Most of us will probably be able to recall at least vaguely that a molecule called ATP is essential for making our bodies move, but this molecule is only a small part of a much larger system. Although we usually aren’t aware of it, our bodies consist of a massive collection of biological motors and related structures, which enable our muscles to contract, nutrients and fluids to move around, and our cells to divide and prosper. Within the biochemical soup that makes up single- and multi-cellular lifeforms, it are these mechanisms that turn a gooey soup into something that can do much more than just gently slosh around in primordial puddles.
There are many similarities between a single-cell organism like a bacteria and eukaryotic multi-cellular organisms like us humans, but the transition to the latter requires significantly more complicated structures. An example for this are cilia, which together with motor proteins like myosin and kinesin form the foundations of our body’s basic functioning. Quite literally supporting all this is the cytoskeleton, which is a feature that our eukaryotic cells have in common with bacteria and archaea, except that eukaryotic cytoskeletons are significantly more complex.
The Cytoskeleton
Although muscles are an obvious example of motor proteins in action, even something as fundamental as cell division (mitosis) involves motor proteins, specifically kinesin microtubule motors. Starting from a centrosome (microtubule organizing center), microtubules are formed from tubulin to create the scaffolding for the kinesin proteins to move across, which then move the two centrosomes (one newly formed) to opposite sides of the cell undergoing mitosis. What drives the actual separation of the duplicated chromosomes (chromatids) are the kinetochores. These kinetochore proteins are microtubule-binding structures that form not only the linkage between the chromatids and a centromere, they also create the mitotic spindle, and which use ATP to ‘crawl’ along the microtubules thanks to their microtubule-binding dynein and kinesin motor proteins. This is what pulls the chromatids apart, allowing mitosis to continue and eventually end up with two sets of DNA within one cell.
In the case of muscle cells, these are rather unique in this regard, as some of them are multinucleated cells, formed through the fusion of individual cells (a synctium). Mammalian muscle tissues come in three broad categories: smooth, cardiac and skeletal muscle tissue, each of which have distinct properties. Of these skeletal muscle tissue is composed of synctium cells, which form long tubular cells, inside of which are many myofibril organelles. These myofibrils consist of myofilaments, each of which can be a thick, thin or elastic type.
The thick filaments are myosin II proteins, the thin filaments are actin proteins and the elastic filaments (titin-based), which provides support and guidance to the thick and thin filaments. Muscle contraction is thus accomplished by the myosin II filaments binding to the actin and moving across it, powered by the ATP from the mitochondria (the powerhouses of the cell). This shortens the myofibril and thus the muscle. Relaxation of the muscle involves the enzyme acetylcholinesterase, which breaks down the neurotransmitter acetylcholine, which originally excited the muscle fiber membrane’s receptors.
Protein Power
Often referred to as ‘cellular currency’, ATP (adenosine triphosphate) and GTP (guanosine triphosphate) are both nucleoside triphosphates, which are an essential precursor to RNA and DNA in addition to being involved in signaling pathways, and the aforementioned energy currency. These nucleosides are generally synthesized inside cells, in the case of ATP using mechanisms like photosynthesis and cellular respiration. While ATP is the most important energy carrier within the cell, GTP is important in DNA transcription and microtubule polymerization, making its use more specialized.
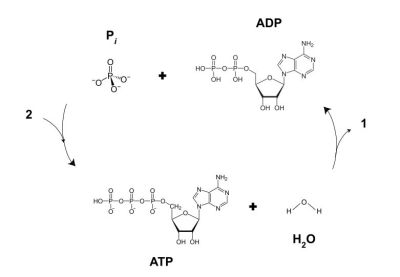
The energy of ATP and GTP is released through hydrolysis, which produces ADP and GDP, respectively, along with a free inorganic phosphate ion (Pi). This releases about 20.5 kilojoules per mole, with the hydrolyzed molecules being ‘recharged’ to produce new ATP and GTP, in a continuous cycle.
For hydrolysis of ATP, the enzyme ATPase has to be present. In the case of e.g. muscle tissue, the ATP will bind to the myosin II proteins, which subsequently gets hydrolyzed by the ATPase, turning it into ADP and Pi. This process forms cross-bridges between the myosin II and actin, which induces movement of the former along the latter, and releasing the ADP and Pi. This is followed by a fresh ATP binding to the myosin II, preparing it for the next power stroke. For different motor proteins a similar process enables a similar process of events, which can continue for as long as it is mechanically possible, fresh ATP (or GTP) is available and an impetus (e.g. neurotransmitter binding to a receptor) is present.
Cilia
Dynein motor proteins move along microtubules, which makes their presence in these flagella and cilia rather logical. These motile cilia and flagella are found throughout the body, with the respiratory epithelial cells found throughout the inside of the respiratory tract providing the essential function of mucociliary clearance, and similar motile cilia moving cerebrospinal fluid inside the brain, as well as egg cells from the oviducts (fallopian tubes) to the uterus .
Meanwhile the version found on sperm cells which provide them with the ability to propel themselves are generally called flagella. This version is longer and has a different undulating motion than the motile cilia described earlier, but still has the same basic structure. As said earlier, eukaryotic flagella and cilia are effectively the same, which has led to considerable confusion and debate in the past.
A Wonder Of Evolution
In this article we touched only upon a fraction of the sheer complexity of all the details which make a body like that of ours work (somewhat) perfectly on a daily basis. Beyond the essentials covered on e.g. Wikipedia, there are the in-depth reference books, with the student reference work Biochemistry (8th edition Archive link) by Jeremy M. Berg and colleagues my current go-to refresher on just about anything to do with biochemical systems.
It should come as no surprise that with the sheer complexity of the field of biochemistry, even something as relatively straightforward as motor proteins would lead to significant confusion. This was quite obvious in a recent video on the Smarter Every Day YouTube channel, where the differences between bacterial and eukaryotic flagella got mixed up severely, which was perhaps somewhat ironic for a science channel that is run by a person with rather strong opinions on ‘intelligent design’ (ID).
The complexity of biological motors is often pointed to by ID proponents as some kind of evidence of ‘irreducible complexity’, yet across the bacterial, archaeal and eukaryotic domains we can see the same problems being solved repeatedly in three very distinct fashions. This shows quite clearly the marvel of evolution, and how this process over millions of years can turn even the most complex problem into a logical series of steps once you get the right chemicals together.
Once the chemistry had some time to turn into proper biochemistry with the evolutionary survival process mercilessly picking off the attempts that weren’t quite good enough, and before you know it you have us primates marveling at at said biochemistry. As they say, life finds a way.
Featured image: “Flagellar Motor Assembly” by [PKS615].
What about converting food waste to energy source with bacteria?
You mean biogas?
Yeast + food waste = ethanol.
Oh, heck, yes… You think someone designed it this way?
“The complexity of biological motors is often pointed to by ID proponents as some kind of evidence of ‘irreducible complexity’, yet across the bacterial, archaeal and eukaryotic domains we can see the same problems being solved repeatedly in three very distinct fashions.”
Macroevolution is based on the idea of incremental change… think of it as the one-by-one, stacking of blocks. If a particular stacking scheme results in advantage, natural selection allows that organism to survive to reproduce, and that stacking scheme proliferates. Add the occasional cosmic ray (a mutating element) and new, more novel stacking schemes might emerge. Over millions of years, an evolutionary stacking organism might conceivably evolve to produce double, triple, or quadruple-stacks, elaborate towers, walls, or pyramids.
There is no way, however, that such a system could ever produce an arch, no matter how many billion years you wait…at least not without external help (in the form of a scaffolding structure, for example). You don’ t need to be a proponent of ID to recognize the glaring holes in the existing macroevolutionary hypothesis.
The fact that nature offers “three distinct fashions” doesn’t support your argument either, it undermines it. What you are in essence claiming is that a hypothetical stacking process incapable of building arches somehow also builds domes and suspension bridges.
Given the supercomputing capability now available, this issue should be resolved, one way or the other. We should be able to simulate a colony of simple one-celled organisms, subject them to time and mutagens, and demonstrate the creation of more complex creatures with multicells and differentiation. If not, why not?
“You will never reach space by climbing successively taller trees”
Local maxima are too easy to fall into for it to have been luck. I wish Hackaday would keep politics out of their articles.
Why is it that mapping the endogenous retroviruses that do and do not show up in different species produces a tree of species that is very nearly identical to the family tree scientists had come up with via observation even before we had the ability to map genes? If a creator did that then he/she/it does NOT want us to know it!
“…wish Hackaday would keep politics out of their articles.”
Hunh? What part of this article has politics in it?
It’s not politics, you’re just wrong. :) It’s not necessary to just naively climb for local maxima using tiny incremental changes; that’s just the basic operation that illustrates the concept. We see in practice that that even incremental changes can at least use various sizes of increment or can have multiple changes per increment. And just making multiple changes or making larger changes is enough on its own to allow you to find all sorts of new minima you would miss otherwise.
But you can also have more complex changes that aren’t necessarily random at all. Maybe one little change is the equivalent of including some old piece of code that was made to do something entirely different, and produces a weird result in the new context. Or maybe you turn something upside down and run it backwards and get some other result. Or sometimes a complex interaction that involves tons of mutually dependent parts can produce a really complex result from a single random spark and a whole lot of prerequisite conditions. Or sometimes it’s possible that things operate by black-magic quantum tunneling stuff. You can and do actually see things reach places you couldn’t reach by just naively hill-climbing, and you can see them when you know intelligence was not involved. And while we don’t know everything underlying biology, we do think we know most of how computing works, and yet computing can produce convincing examples these sorts of behaviors too. So it seems far more likely that it’s a property of various systems and the cumulative efforts of tons of experts have some merit, instead of thinking than that biology is a special mystery.
Stone arches and domes and plant suspension bridges are actually quite common in nature, without any intelligent intervention.
As for simulations showing evolution towards more complex structures, we’ve been doing that for decades.
“As for simulations showing evolution towards more complex structures, we’ve been doing that for decades.”
Really? Please cite one credible simulation/study showing that random mutation results in greater complexity–I’m not talking about white pepper moths to brown pepper moths, or short bird beaks to long bird beaks. I’m talking about a single-celled organism becoming a fish, a fly, or a mouse. I’m not arguing with you. I’m asking for the material so I can look at it myself.
The notion that a thousand monkeys typing on a thousand typewriters for a thousand years will eventually result in Shakespeare is not accepted by anyone who understands probability or mathematics.
“Stone arches and domes and plant suspension bridges are actually quite common in nature, without any intelligent intervention.”
First, I took no stand on “intelligent intervention.” Second, if your take-away from my earlier comment is that nature can’t produce stone domes, I’m afraid you completely missed the point.
If you stipulate a system of self-replicating machines whose features are predicated on random incremental change, and one where natural selection conveys advantage only to those mutations/changes that improve a creature’s survival, how then an you get from creature “A” to creature “E,” if the intermediate mutation states “B,” “C,” and “D” don’t confer any survival advantage?
That was the point I was making with the incremental building-block analogy. You can build all kinds of things by stacking blocks upon each other… towers, walls, pyramids. But without a transitional state (during which the blocks are not viably self-supporting) you can’t build an arch. So what provides the “support” or “scaffolding” during that transitional state?
This is not a political question. It’s not a religious question. It’s a question that any legitimate biologist should expect to hear and have a plausible answer for. “We simply don’t know,” by the way, is an entirely acceptable answer. Magic hand-waving and “Blah blah blah… billions of years…” is not.
Except any legitimate biologist will tell you we actually do know. Evolution has been the consensus of the scientific community for a long time. We’ve observed evolution in real time over the decades since Darwin, and the fossil record makes sense. Even modern genetic testing has reinforced evolutionary models that were previously based on morphology alone. It’d be like saying archeologists don’t actually know where Ur was since we can’t witness it being built and are just guessing based on archeological evidence. There’s more than enough evidence to be sure. Find me a “legitimate biologist” who denies evolution exists.
As for simulations, my dude I was writing my own genetic simulations to evolve life in physics sims over 20 years ago, and I learned it from a book published in the 80s I got from a library sale. It is a nice project for any highschool student. BreveCreatures was a big inspiration. Google “genetic algorithms” and you’ll find a ton of simple examples to get you started.
We can’t really understand everything that happens in evolution in the scale of billions of organisms, each consisting of billions or trillions of cells. Similar to how we cannot quite understand large neural networks – the output is more than what you would expect to get by just looking at the structure and the training set.
But then again, maybe there is some fundamental mechanism that we don’t yet know anything about. It doesn’t have to be any “intelligent design” entity though.
You probably aren’t aware but your simplified model of evolution is a strawman. Sure… the occasional point mutation isn’t enough. No shit Sherlok. Go learn about jumping genes and endogenous retroviruses. That’s just a couple of tools evolution has in it’s kit besides just simple point mutations. And as you learn all these new things, remember… that’s just the simplified version for us lay people. Real genetics and evolution are something you could devote a lifetime to learning about. And some people do.
A purely artificial and deterministic system, such as a computer simulation, can easily be made to give the impression that an impressive and complex structure has appeared out of noise. All it takes is to repeatedly process the input in such a way that changes which make it appear more structured are favored. It’s not intuitively obvious that what looks like chaos to us is capable of that, but it is. That’s a condensed view of the basis of “AI” image generation. I do us a disservice by leaving out the fact that the way we make those is by feeding it lots of existing images instead of writing a set of rules from scratch that somehow expresses what we do and don’t consider meaningful.
But other less intuitive things show the same effect. The times we’ve taught an “AI” to do other things purely using reinforcement, such as when they are made to learn to drive a virtual racecar around a track or to walk or to design an antenna with various characteristics, we just feed them random noise and reward the best results from generation to generation and end up with perfectly functional results after awhile without ever actually stepping in to intelligently design their behavior other than imposing constraints and rewards and such.
Or there’s even quantum annealers, which can find the global max/min of a complex system we set up. Again they operate without intelligence input once the constraints / system is defined. They basically work by making it so that they could take on any valid state but will tend to take on the one that is most stable, which is the solution. Oh, and converging solutions as a general task, even outside of quantum hardware, is something where you can jump into the global min/max much faster once you have enough energy/noise to not spend all your time slowly climbing smaller hills. Sort of an activation energy effect. The tunneling dodges the barriers, but noise and reinforcement works too – where the strength of noise has to be enough to reach the better state, or else you devolve back into hill climbing.
Even though it takes a bit more to make life than to tell a racecar how to speed up, slow down, and turn, there’s also a lot more time and space in which possibilities can be evaluated; the entire earth instead of the surface of a few square centimeters of silicon. And there’s a lot of kinds of interaction and feedback that can occur that may make a given solution even easier to reach than if we could only naively change one thing at a time and then re-test.
Or to put it another way, maybe it seems dubious to a layperson working on intuition, but it checks out once you really study it.
I see here two problems with your reasoning:
– you made analogy to explain the concept and than by showing fault in analogy try to prove concept being wrong.
– You can put two blocks nearby with third on top and you get “proto-arch” (even a “proto-scaffold”!) that once sealed with two other blocks will form “proto-dome”. If your environment promotes tall archs – just two towers going up and drifting toward each other. I admit though that suspension bridge is beyond my Jenga-Fu.
Arches National Park.
Plants like forsythia bend over as they get taller. Eventually the longest branches touch the ground, and after a while they’ll take root. Voilà: an arch.
Been demonstrated times and times again, for decades – see Avida and similar a-life simulations.
See the curious stacking patterns in slime mould for example – we have quite a good understanding of the underlying mechanism now, and it’s quite simple despite its ability to produce very complex behaviour. Evolution always finds a way. Hacky, convoluted, hilarious way, but it works. Sort of.
After watching Dustin talk at the end about the flag-planting, I noted that it happened just after he briefly talks about how this could have happened via small change (i.e. via evolution) and mentions this injection structure (that is perhaps older and simpler?) that it may have developed from.
In that context, it is almost like he is talking to himself and slowly giving himself permission to move his flag from the God/ID camp to the evolution/science camp. It will be interesting to check back in in a few years if he continues to keep abreast of this highly-detailed biological science.
I interpreted that as him giving himself an excuse to promote ID on his science channel.
No – the fact that we can understand any of this is the hack.
Oh for the love of Christ.. Inspiration is also important, it’s mildly interesting. Who cares
I think it’s incredibly unprofessional to bring up ID in this article.
Just stick to the science and current scientific belief. Even if your opinions on ID align with current scientific belief, it’s still very inappropriate to go out of your way to bring up religious concepts just to tear at them.
Hard disagree. It is essential to tear at wrong and unscientific ideas like ID, even/especially if those ideas try to cover themselves in a thin veneer of religiosity.
There is nothing in the universe that can create the universe. According to our current understanding of physics, the universe had to have originated outside of the universe. Whatever that thing is outside our universe, it does not have to play by the same rules. At that point, the question of whether that thing created the universe on purpose or by chance is a valid and rational query.
@Jace
What if the universe was always here? Nothing needed to “create” it. Besides, if you start talking about creators, you end up in infinite regress anyways.
@Anonymous
An equally valid inquiry. This was called the “Steady State Universe Theory” and was actually a very popular opinion for a very long time. The “Big Bang Theory” was originally named so by people as a way to mock the theory when it was first proposed.
To your second point:
Our universe, as we understand it, does have to have an origin point. Something outside our universe might not have such restrictions, so that would not result in infinite regress.
I’m not making a religious argument. I’m merely stating the fact that something other than the universe had to have started our universe.
So, for these motors, can someone give us a torque constant (N*m per amp) or other figures that would let us compare and contrast against electric motors? Maybe there’s a lesson to be learnt for adapting the principles of these motors in to something a bit more macro-scale?
Since it is not an electrical motor, I’m not sure how to get the equivalent torque constant, but here are some numbers I found, for your perusal.
Max torque : ~200 pN.nm
Power usage: ~1pW
Efficiency: ~100%
Another interesting statistic, 20.5 kilojoules (as in 20.5 kilojoules per mole of ATP) is the energy in about one sip from a soda, or a bite of a cookie, or burning a 100W lightbulb for 3.4 minutes. (courtesy of ChatGPT).
https://www.pnas.org/doi/10.1073/pnas.1501734112
https://www.ncbi.nlm.nih.gov/pmc/articles/PMC5115386/
Heck yeah!
Was thinking the same thing!
That’s the shibboleth for “not AI generated”
So basically, life only needs time and resources to keep going; no need for any kind of “divine things.” Sadly, you can’t debate with religious people anymore without bumping into walls like “God loves you even if you don’t believe” or “there was a designer,” or even worse if you talk with evangelicals, Jews, or Muslims. And please don’t take it wrong; I have nothing against them. I understand the roots of faith and why it is a good thing, but the dark side is that most religious groups segregate non-believers, and that puts you at a certain disadvantage in a social context. Annnndd we have the new beliefs of younger people: angels, energy, crystals, multiple dimensions, inner gods, youtube gurus, you name it. :)
Clearly our progenitor was Shub-Niggurath.
Well ,we are lucky, we didn’t get an octopus-like face!
Ok, no divine things. What do we get? The beliefs of younger people. But does that make them free? They do not believe nothing, they believe everything (don’t know who said that first). Why? Are these beliefs better?
So, if there is a god who wants us to be really free, he must not be scientifically proofable, otherwise we would not any more be really free.
That said, I don’t see the contradiction between creationism and evolution, because evolution is a really good way to create without being proofable.
Some points. Don’t believing is OK. Believing something doesn’t mean it is exist or is true. It is OK to accept we don’t know something, (that is how we make science btw).
Evolucion is a cientific theory, it can be false or true, on both conditions science wins. creationism in the other hand, it make gods from designers or creators.
freedom, the problem with freedom is that we use ther word in a very subjetive way, freedom is a different thing for each person, some extreme conditions can spot where there is no freedom of course, But usually freedom is a fussy thing. So going to the basic human condition to try to check if we are free on not, I think was Buddha (I can be wrong!) who said the 3 destinies of a human being are living, illness and death, in a sense we are condemn to one of them no matter what(I guess I can add taxes:). So I can easily said that freedom only can occur if you are out of the scope of the 3 destinations.
About gods and religions, lets put an example, would you have faith and accept that right now , there is a big circular grow in the dark big rock buried 3 feet below exaclty in the place you are standing? even better, the people who said that lived 3,400 and 1,900 years ago and had no idea of what a scientifi method was? they wrote it down ,but during the next years the text was changed, and changed and…
so from now on, the only way to know the true is… digging 3 feets of course :)
yes, but if something is true or exists, it is reasonable to also believe it (taxes for example :-)
As I said, you can prove or disprove evolution, but that contains no information about creationism. You would have to proof that no divine entity is able to use evolution as tool.
So it is not basic. My start point is, for any kind of freedom you have to be able to choose in that matter. If you can prove or disprove something, you are no longer free, or backwards: freedom requires the absence of a proof in respect to the subject in question. That does not mean there are no other conditions which may cut down freedom.
guys how do you make the citation thing here in HaD?…
–As I said, you can prove or disprove evolution, but that contains no information about creationism–
I agree with you mostly, ideally a theory about creationism should be elaborated so we can prove or disprove.
—yes, but if something is true or exists, it is reasonable to also believe it (taxes for example :-)—
Yeah I agree with you, we also “believe” in inmaterial or abtract concepts, like institutions, goverments, even fiat money and cryptos so yeah.
—-You would have to proof that no divine entity is able to use evolution as tool.—-
I cannot agree with you. Actually I think is should be the opposite, they would have to proof that a “diviny entity” exists in the first place. You see, the posibility existence of a god that designed life is really problematic, not for the scientists, but for the believers, lets imagine for a second that science found evidence of a god making life from scratch. Ok, now what? that brings the astonishing posibility of not only one god (sorry monotheism!) but more than one! so which one make the life as we know it? we know a couple of candidates in this side of the world, but what about the others? what if all religious people is wrong and in some futuristic and optimistic way we find that Aton was the lucky one ? Shub-Niggurath doesn’t count :)
So interesting talk Matthias, but I’m afraid Jason thinks it’s inappropriate to bring up religion in an article :( , personally I think even religion is hackable.
just like in the good ol’ usenet, a leading “> ” (I discovered by chance that these two characters are recognized by the comment software)
I think we agree here: to prove intelligent design you have to prove the existence of a divine entity first (or get that proof as corollary). Would be interesting, what the proof says about the number of gods :)
But this proof would probably not verify or falsify evolution theory as such, as well as the proof or falsification of evolution would say nothing about the possibility of intelligent design (which may or may not use evolution as tool). So I can’t see the contradiction evolution vs. design.
ok, let’s talk about mathematical logic instead ;-)
Freaking code reuse! Sheesh. Oh look at that elegant and robust code. It’s so effective we might as well repurpose it and adapt it to another application rather than gen up some novel solution multiple times. Maybe we can call this well designed. But certainly not intelligent design.
Just adding that I found that this was an intelligently write up of the topic though I am an ignorant lay person. The only fault that I find with it is the gratuitous swipe at a religious person and intelligent design. His error has no relation to his beliefs as to origin narratives. Since when was “life does not arise from non-life” disproven with a reproduce falsifiable experiment?
The problem is when the repository contains something really handy like Taq polymerase, but the only critter maintaining it occupies an evolutionary dead end in some hot spring with liberal concentrations of dissolved heavy metals.
I think it’s inappropriate to bring up religion in an article.
Sorry for that :( I was trying to pointing out that it would be great that religious people can read and understand this kind of studies.
Tell that to Destin!
Now to attach several thousand of them to a large sun gear and get them to turn a magnet.
Maybe make a stand of DNA that can print out protein generators on mass.
What a pity that those last two paragraphs were added. Just read them aloud for a bit, and notice how there is absolutely no rebuttal of ID, but rather the repetition of a mantra; the droning up of the same story with actual no defusing power at all.
As James Tour said, if we really want to start doing origin of life research, we must first let go of theories we know are a dead end.
Hello Elliot and Maya. Do you remember a short time ago you(Elliot) wrote about “If you can’t say anything nice….”. I’m wondering if you think Maya’s statement singling out Destin with
“This was quite obvious in a recent video on the Smarter Every Day YouTube channel, where the differences between bacterial and eukaryotic flagella got mixed up severely, which was perhaps somewhat ironic for a science channel that is run by a person with rather strong opinions on ‘intelligent design’ (ID).”
is an example of your previous statement that “What all of these comments have in common is that they’re negative, low value, non-constructive, and frankly have no place on Hackaday.” When I remove Maya’s statement above, it does not remove anything of value from Maya’s otherwise wonderful article on biochemical motors. Also, I understand Destin to be an engineer geek, like a whole lot of us, not a Lettered Biochemist publishing in a reputable scientific journal.
I don’t think mentioning ID as having some flawed reasoning is the problem; it was the singling out of Destin’s beliefs and misunderstandings that seem personal and unconstructive. Why not go after the ID Scientist with letters who do publish, rather than a Youtuber? There are quite a few of them.
Oh, also Maya, your last paragraph contains three words that introduce a ghost in the biochemical machine: “mercilessly” as if random mutation can ever be merciful; “attempts” as if random mutation can be willful toward some purpose or goal; and “finds” as if evolution is searching for a better solution to something that isn’t good enough. Kinda letting the camel’s purposeful nose in the tent.