Despite being present in everything that contains water, tritium is not an isotope that many people were that familiar with outside of select (geeky) channels, such as DEF CON with a tritium-containing badge, the always excellent NurdRage’s assembly of a tritium-based atomic battery, or the creation of a tritium-phosphor-based glow-in-the-dark tesseract cube.
Tritium is a hydrogen isotope that shares a lot of characteristics with its two siblings: 1H (protium) and 2H (deuterium), with the main distinction being that tritium (3H) is not a stable isotope, with a half-life of ~12.32 years that sees it decay into 3He. Most naturally occurring tritium on Earth originates from interactions between fast neutrons (>4.0 MeV) from cosmic radiation and atmospheric nitrogen.
Recently tritium has become a politically hot topic on account of the announced release of treated water at the Japanese Fukushima Daiichi nuclear plant. This has raised for many the question of just how much tritium is ‘too much’ and what we’re likely to notice from this treated — but still tritium-containing water — being released into the ocean.
On Becquerels and Equivalent Dose
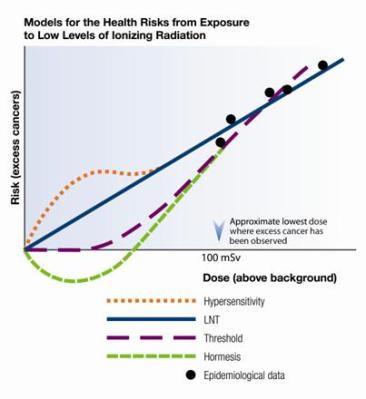
When assessing the risk of exposure to radioactivity, the Linear-Non-Treshold (LNT) model is most commonly used. This model essentially claims that there is a perfectly linear match between exposure to radiation and the chance of developing cancer and other negative side-effects that could be caused by said exposure.
As has become apparent through multiple recent studies, reality isn’t that simple. Between the varying effects of different types of radiation on different parts of the body and the role of the body’s ability to repair damage to cells, the reality is that we see an effect far closer to that of what is often called radiation hormesis. This is the theory that in low doses, radiation can even be beneficial.
Far-fetched as this may seem, studies such as e.g. Bannister et al. (2016) and Khan et al. (2021), on mouse models showed no cytotoxicity or genotoxicity in the spleen after exposure to beta radiation from tritium (Bannister et al.), or found that the immune system had been upregulated after exposure to low-dose radiation (LDR, Khan et al.). As noted by Khan et al., the current evidence in the literature points to LDR being a positive influence on the body’s immune system, which could have significant effects on our understanding of e.g. cancer treatments.
These kind of studies also give us some idea of how afraid we should be of e.g. the radiation in wine, as studied by Tonev et al. (2018). Here the levels of 137Cs, 40K and 3H are used to try and pinpoint the age of wine, to help identify wines that are being passed off as a much older vintage. Their findings for Melnik (Bulgaria) wines from a 2001 vintage were 137Cs levels at <0.15 Bq/L compared to 44.6 Bq/L in 1986 due to the fallout from the Chernobyl power plant’s #4 nuclear reactor.
Meanwhile, 3H levels fluctuated between roughly 7 to 63 Bq/L and natural potassium-40 (40K) between 15 to 20 Bq/L over that same timespan. We can compare this to the natural 3H levels in the atmosphere and surface water using information provided via the Canadian Nuclear Safety Commission (CNSC) as part of its tritium studies. Expected tritium level in rainwater is about 0.6 Bq/L, while surface waters range from 0.37 to 1.11 Bq/L.
Atmospheric nuclear weapons testing has elevated environmental tritium levels between 1945 and 1976, with an estimated 1.7 x 1020 Bq tritium produced. This tritium would have gradually made its way out of the atmosphere in a manner similar to natural (cosmic ray) tritium and ended up in surface waters and from there into organisms and ground water.
At the Fukushima Daiichi site, roughly 860 TBq (0.86 x 1015 Bq) of tritium are stored, diluted across many liters of water. The tritium itself is part of so-called tritiated water (HTO, T2O, or super-heavy water), which is chemically virtually indistinguishable from H2O and D2O (deuterium-containing heavy water), which is why it isn’t easily separated from the H2O. Heavy-water based Canadian CANDU reactors (HWR) in normal operation release about 430 TBq per year, while a PWR like the Westinghouse AP1000 releases about 20 TBq/year and a BWR about 1 TBq/year.
Considering that the tritiated water at Fukushima Daiichi will be released gradually and heavily diluted with seawater over the course of years, this should make it obvious that the amounts being released are insignificant compared to that released by just the active nuclear plants around the world. So nothing to worry about, then?
Oceans Are Big
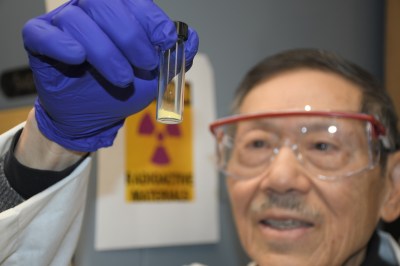
With roughly three-quarters of the Earth’s surface covered by water, there is a lot of water to dilute something like tritiated water into, as well as e.g. uranium, with PNNL reporting recovering 5 grams of yellowcake (uranium) from seawater in 2018. It is estimated that there are at least four billion tons of uranium in seawater, diluted to about 3 parts per billion, which at some point in the future could become economical to recover.
When it comes to e.g. drinking water, we have come to accept that there will always be some amount of heavy metals, minerals as well as radioactive isotopes (e.g. radon, uranium, and tritium) in it. This is why countries have set limits on what amounts are deemed acceptable in drinking water. For tritiated water, this varies heavily, with Australia accepting over 76,000 Bq/L, while Finland aims for 100 Bq/L, or roughly five liters of Melnik wine worth of 40K equivalent.
An interesting point worthy of note here is that potassium-40 behaves just like its stable potassium forms when it comes to its biological role. As a result, an adult human body that weighs 70 kg will contain about 140 grams of potassium. With 40K naturally occurring at a rate of 0.0117%, this means roughly 0.0164 grams of the isotope and ~4,300 Bq worth of beta decays per second.
Since 3H and 40K are both beta emitters, but only potassium bioaccumulates in any significant manner, this would theoretically make eating a banana riskier than drinking tritiated water, even at a relatively high levels of HTO. Not unexpectedly, at the WHO-recommended level of 0.01 MBq/L in drinking water, Guéguen et al. (2018) reported no noticable physiological effects on tissues in mouse models.
Lower Is Always Possible
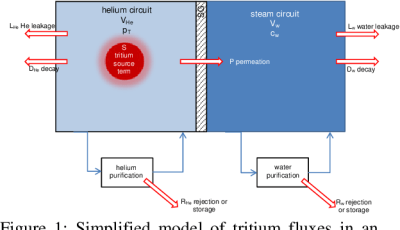
The production of tritium is not a goal in nuclear reactors, but occurs as a side-effect, with pressurized water reactors (PWRs) using boric acid as neutron poison in their primary loop to help with moderating the nuclear chain reaction. Boron-10 (10B) can sometimes capture a neutron and produce 4He and 3H. Similarly, in heavy water reactors (HWRs) like the CANDU, deuterium will also capture neutrons and turn into tritium.
Most of the tritium produced in the reactor’s primary loop remains there and is at some point removed and used for commercial and other applications. Since tritium is a hydrogen isotope, it also has that other amazing feature of hydrogen: the ability to ignore attempts to contain it. Much like how containing 1H is a problem due to hydrogen permeation, so too do nuclear reactors have the issue of containing the tritium inside the primary loop. Fütterer et al. (2016) cover this issue in detail for upcoming Generation IV high-temperature reactors, which use helium as coolant.
Generally, the point where some tritium permeates into the secondary loop is via the heat exchanger. These are devices where heat transfer efficiency is important, which means thin (nickel-alloy) walls. Although resistant to embrittlement from hydrogen diffusion, these heat exchangers do allow some hydrogen to permeate through, which is what ultimately ends up at the turbines and in the cooling water that is either released into a nearby body of water, or to the atmosphere in a cooling tower.
Since HWRs and PWRs generate a fair amount of tritium in normal operation in their primary loop, this means that relatively more hydrogen (thus tritium) will permeate into the secondary loop. New alloys for the heat exchanger may reduce the amount of permeated tritium in the secondary loop, or more effective capture mechanisms may enable even the low amounts of trititum in the secondary loop to be filtered out.
All of this will also be important with future nuclear fusion reactors, which will generally use a deuterium-tritium (D-T) fuel mixture, as well as with the increased use of hydrogen in industrial and other applications. Containing hydrogen isotopes is essential, regardless of whether it is merely a waste product as in fission reactors, an ingredient in industrial processes, a fuel, or an energy carrier.
A Radioactive World
Perhaps the most harmful part of the LNT model is that it creates the illusion that a world with zero radiation is somehow possible, or at least highly desirable. This is where statements in the media about ‘TBqs worth of tritium’ without further context and a distinct void when it comes to interviewing e.g. physicists and other experts in the field (like those at the IAEA) are anything but helpful.
While the release of tritium by nuclear plants and other artificial sources (like discarded tritium-based batteries and self-illuminating signs) is undesirable and something that needs research into ways to further limit its impact, it is not a significant issue. We live, after all, on a planet that itself is radioactive, sheltered by an atmosphere that for the most part keeps us safe from cosmic rays and other radiation hazards.
As with most things in life, the key to happiness lies in finding the right perspective.
[Heading image: Fukushima Daiichi at night. (Source: Tepco)]
“All of this will also be important with future nuclear fusion reactors, which will generally use a deuterium-tritium (D-T) fuel mixture”
“Critical” is more like it. Fusion reactors will generate their own tritium via breeder blankets, and they’ll have to master separation. Have to. If my math’s right, a fusion reactor would deplete the entire Earth’s supply of natural tritium in about 2 weeks, and the supply of man-made tritium easily in a year (more likely a month).
A fusion reactor would also dramatically change the tritium (and helium-3) economic market. It’s been pointed out, for instance, that the tritium/helium-3 that a fusion reactor would produce could actually be worth more than the electricity. By, uh, a lot.
What is a CURRENT going-Rate in $ of Tritium and Helium3 at the moment?
Found something:
https://www.lpi.usra.edu/decadal/leag/DecadalHelium3.pdf states 1’400$ per gram for Helium3 (Pricetag @ 2017-Levels)
and for Tritium @ 30’000 $ per gram (Pricetag @ 2000) in a paper from Los alamos https://fire.pppl.gov/fesac_dp_ts_willms.pdf
Pricey stuff indeed!
Yeah, and even those prices are artificial because of the supply. The market’s basically entirely closed, which means in some sense the “price” is just however much the US government feels like charging you. If Jeff Bezos comes to the DOE and says “100k liters of helium-3, how much?” they’d make him pay for expanding production (so it’d be *way* more).
For helium-3, for instance, the DOE’s pushed hard to try to stabilize the market by reducing its demand – the market nearly exploded in the early 2010s. If the supply increased dramatically, it’s not actually a guarantee that the price per liter would immediately go down.
We pay about £10k for 5 litres of 3He at atmospheric pressure.
Are you going to make me pull out Avogadro’s constant to see how that compares to the price Thomas cited?
Such a shame that the various fusion research centers in Japan, Canada, or the U.S. cannot find a use for a large amount of tritium-enriched water, or that no one in Japan needs a light source with 25-year life span.
Canada doesn’t need tritium, it comes out of our reactors.
Tritium is also critical to modern “miniaturized” nuclear weapons which is why an A-bomb or fusion trigger the size of a bowling ball is possible, which in turn is why H-bombs are small enough to fly on missiles. This is a major reason such weapons must be periodically serviced and stop working after awhile if they aren’t. (The exact interval at which they stop working is a closely guarded secret, but probably is probably comparable to the half-life of tritium.)
Intuitively, I expect leakage out of container to be faster than the nuclear decay.
It is probably a good practice to use consistent units. The article quotes a WHO recommendation of 0.01 MBq/l, which looks tiny. When written as 10000 Bq/l it’s easier to compare with national recommendations: 100x larger than Finland’s, but 7.6x smaller than Australia’s. I think different national recommendations reflect the local environment; Australia is mineral-rich, so the natural background is probably higher and maybe the ‘organic’ water is naturally radioactive.
I don’t know at which point it’s still safe to drink such radioactive ‘organic’ water, and at which point one should say “don’t touch this thing'”, which is of course true for other organic things as well—as the article points out, everything should be taken in context; it’s possible to overdose on natural supplements, and on pure water.
I reckon the finnish recommendation is formulated based on the high radon gas levels in southern Finland, among the highest in europe.
The solution to pollution is dilution.
unfortunately not, because inconvenient things like fish like to accumulate it and a lot of things and humans like to eat fish
Tritium doesn’t bio-accumulate because it doesn’t function in a biological role – like deuterium water, it’s different enough that cells can’t use it. The half-life in the human body is 7 – 14 days. A person normally loses about 2-3 liters per day and the average person has about 55 liters of water in their body, so the time is consistent with mixing and excretion: it simply goes through you.
The backlight of my Sensor TriLite digital watch (circa 1980) could use a Tritium recharge.
I had one of those way back then. Thought it was magical. Then the new puppy chewed it up. Was very disappointed she did not glow
the phosphors would need to be changed as well, the constant electron bombardment slowly damages them…
> The tritium itself is part of so-called tritiated water (HTO, T2O, or super-heavy water), which is chemically virtually indistinguishable from H2O and D2O (deuterium-containing heavy water), which is why it isn’t easily separated from the H2O.
H2O 0.9982 Density at STP (g/mL) ; melting point 0.00 °C; 32.00 °F; 273.15 K
HDO 1.054 Density at STP (g/mL) ; melting point 2.04 °C; 35.67 °F; 275.19 K
D2O 1.1056 Density at STP (g/mL) ; melting point 3.82 °C; 38.88 °F; 276.97 K
T2O 1.21 Density at STP (g/mL) ; melting point 4.48 °C; 40.06 °F; 277.63 K
Since heavy water sinks to the bottom of the liquid when it forms ice, I assume that Tritiated water does as well ? Anyone know ?
If it does then just lower the temperature to 0.1 °C hold it there for a year and whatever sinks will be either radioactive tritiated water(T2O), Heavy water(D2O), Semiheavy(DHO) water and the mostly Light water (H2O) will be the liquid floating above the solid mass ?
Thermal motion will keep diffusing it around too much.
The idea that heavy water is chemically indistinguishable is bunk. The mass of the nucleus has a large effect on the chemical bond energy, so it behaves quite differently especially in biological systems that rely on fine tuned hydrogen bonds. Some bacteria can live in 98% heavy water, but 50% heavy water becomes lethal to most other organisms because cells stop dividing in it.
The night sights on my pistol contain tritium.
He ain’t heavy. He’s my brother
Win!
File this under under “chemists create ideas for Adam Sandler sequels” Vin Diesel plays Adam Sandler’s long lost brother, we’ll use CGI to make him look out of shape at the beginning and a training montage to return him to his normal look. Title the whole thing “The Heavy Waterboy”. And if the line “That’s some high quality T2O” doesn’t get worked into the whole thing, then a script writer’s going to discover their h20 was secretly replaced with h2so4.
Whenever I hear again about hydrogen permeation, I recall how excited technologists are thinking of “green” hydrogen economy, and I worry. Using pure molecular hydrogen at industrial levels is bound to spend significant amounts of Earth’s water, probably in matter of few centuries! Released, leaked hydrogen (ditto for helium, but we don’t need it as much) is lost from atmosphere into interplanetary space very soon.
Atmospheric escape of hydrogen is certainly real, and Earth will likely have no oceans remaining in a billion years due to a combination of tectonic subduction and an increase in evaporation (and hence atmospheric loss) due to a brightening of the sun. But I think you underestimate the enormous amount of water on the planet. According to Wikipedia, approximately 87 million tons of hydrogen gas was produced in 2020. If *all* of that hydrogen were lost to space every year, it would be equivalent to losing around 700 billion liters of ocean water annually. At that rate it would take about 2 billion years to drain the oceans. If we increased it to the equivalent of a million liters of water lost to space every *second*, it would still take about 45 million years to drain the oceans. Of course a reduced sea level might be a problem long before that, but I think it’s safe to say that if humans want to survive as a species long enough for lack of hydrogen on earth to become a problem, there are a whole lot of other problems we’ll have to solve first.
Surely a large percentage of it would combine with oxygen when passing through the ozone layer (UV-C from the local star splitting O2 is what forms the Ozone layer) and then be pulled back down to earth by gravity due to its increased mass.
The bond dissociation energy for oxygen (O=O) is 5.15 eV (~240.7nm)
The bond dissociation energy for hydrogen (H-H) is 4.52 eV (~274.3nm)
So UV-C energy from the local star should form some water modules from the leaking hydrogen as well as ozone. But as for the Helium it is gone forever.
one tiny note – most of industrially made hydrogen is from natural gas, not water ;-)
“Boron-10 (10B) can sometimes capture a neutron and produce 4He and 3H.”
?
I read that slow (thermal) neutron capture gives 7Li and 4He.
Then the 7Li can capture a fast neutron to give 3H and 4He