You might think that particle physicists would be sad when an experiment comes up with different results than their theory would predict, but nothing brightens up a field like unexplained phenomena. Indeed, particle physicists have been feverishly looking for deviations from the Standard Model. This year, there have been tantalizing signs that a long unresolved discrepancy between theory and experiment will be confirmed by new experimental results.
In particular, the quest to measure the magnetic moment of muons started more than 60 years ago, and this has been measured ever more precisely since. From an experiment in 1959 at CERN in Switzerland, to the turn of the century at Brookhaven, to this year’s result at Fermilab, the magnetic moment of the muon seems to be at odds with theoretical predictions.
Although a statistical fluke is basically excluded, this value also relies on complex theoretical calculations that are not all in agreement. Instead of heralding a new era of physics, it might just be another headline too good to be true. But some physicists are mumbling “new particle” in hushed tones. Let’s see what all the fuss is about.
The Electron’s Big Brother
Muons have a spin and charge that creates a magnetic moment, which measures the strength and orientation of its magnetic field. You can imagine muons like tiny permanent magnets whose orientation aligns when they are placed in an external magnetic field. The “g-factor” is a dimensionless proportionality constant between the magnetic moment and the spin.
Using some fancy math, one can calculate the g-factor for muons (or electrons) from the Dirac equation, which gives a value of exactly 2. But this is not the whole truth: in quantum electrodynamics (QED), “virtual” particles can pop in and out of existence, so long as they do so quickly enough that they fall under the cover of the Heisenberg uncertainty principle. This in-and-out-of-existance loop leads to correction terms in the calculations. For the muon, loop corrections lead to a g-factor that is not exactly 2 but 2.00233183620, and the difference is referred to as the anomalous magnetic moment of the muon.
All You Need Is a Giant Magnet
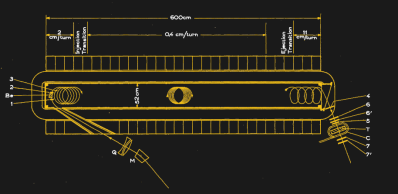
Credit: G. Charpak et al.
In 1959, CERN decided to run an experiment that should test the validity of quantum electrodynamics, which predicted the anomalous magnetic moment of the muon. Ignoring the usual tradition to come up with a creative acronym, the experiment was simply called “g-2” (pronounced “gee minus two”).
In the experiment, a beam of protons from CERN’s synchrocyclotron was shot onto a target to produce a beam of pions that immediately decay into muons. This muon beam then entered a six meter long magnet.
The magnetic field was oriented vertically to the muon beam which caused the muons to curve into a circular path. The magnetic field also varied from the left to the right, which was achieved by carefully inserting exactly calculated shims into the magnet. This caused the muons to slowly drift from left to right making a spiral curve.
In this magnetic field, the spin of the muons is wobbling (precessing) just like the spin of protons in an MRI machine. At the right end of the magnet, the muons are ejected and the direction of their spin relative to their momentum is analyzed. From this measurement, the anomalous magnetic moment can be calculated because it is sensitive to the difference between the orbital frequency and the spin precession frequency. Only six months after the start, the experiment came up with a result of g = 2.001165±5 which agreed well with the theoretical value at that time and thus confirmed the validity of QED. In the coming years, a second experiment with 25 times better accuracy actually found a difference between theory and measurement, but this vanished after theorists refined their models. A third and final experiment at CERN confirmed this new theoretical results with an astonishing accuracy of 0.0007% (7 ppm).
The Tension Between Theory and Experiment
In 1984, the US took over in investigating the muon anomalous magnetic moment. Using the proton accelerator at Brookhaven National Laboratory (BNL) their experiment introduced several improvements over the CERN measurements. These included a higher proton intensity, a 14 m diameter superconducting magnet — the largest in the world at that time — more efficient muon injection, and electrostatic focusing of the muon beam as well as custom-made, 400 MHz waveform digitizers. The aim of the experiment was to achieve an accuracy of 0.35 ppm in order to check the loop corrections caused by W and Z-bosons which were discovered the year before at CERN.
To obtain an objective result the collaboration used a “blind analysis”. This is a common technique used in particle physics to avoid the unintended bias towards a certain result by the people analyzing the data. It is similar to the double-blind randomized clinical trial used in medical research. In the BNL experiment, the data analysis to obtain the two frequencies from which g-2 could be calculated was carried out by two different teams. In addition, each frequency had an artificially introduced offset which was unknown to the data analysis team and was only subtracted after the result had been fixed.
When the final data-taking run ended in 2001 the combined result was in disagreement with theory by 2.2 – 2.7 standard deviations depending on the theoretical calculation. This caused much discussion of whether it could be a hint towards new physics because yet undiscovered particles would lead to additional correction terms to the theoretically calculated value. As with every other result, there is of course the less spectacular possibility that it is merely a statistical fluctuation which at 2.7 standard deviations is unlikely, but as history has proven not at all impossible. Another boring explanation would be some unaccounted systematic error in the experiment.
And the theoretical value is not itself bulletproof. This is because there are large corrections from particle loops that involve strongly interacting particles that cannot be calculated from theory. Instead, theorists use measured production rates from other experiments for these particles to approximate the correction term.
An Eagerly Awaited Result
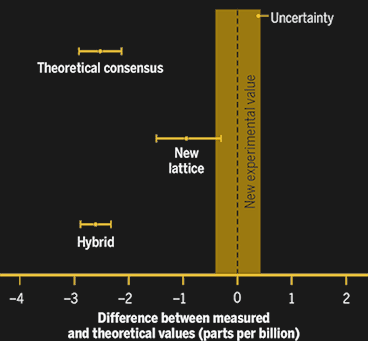
Credit: V. ALTOUNIAN/SCIENCE
In 2013, the superconducting magnet of the BNL experiment was transported 3,200 miles to Fermilab near Chicago in order to repeat the experiment using their more intense muon beam. The result was eagerly awaited by the physics community as the tension between theory and experiment had been standing unresolved for the last 20 years.
A few weeks ago Fermilab published their first result that was again obtained by a blind analysis where the frequency of their main clock was entrusted to two other physicists outside of the collaboration. After unblinding the data, it became clear that the result confirms the BNL measurement.
The combined tension between theory and experiment amounts now to 4.2 standard deviations which is just a bit short of the 5 standard deviation threshold to claim a new discovery. Still, the difference between the theoretical and experimental values is large enough that the chances of it happening randomly is about 1 to 40,000. An experimental screw-up is also unlikely, now that the result is confirmed by two independent experiments, even though they use the same technique and some of the same equipment.
Ironically, the theoretical value might be to blame. In fact, on the same day that Fermilab published their result, a new theory paper published in Nature, but already available since last year as a preprint, arrives at a value that is actually compatible with the experimental data. This new theoretical value was completely calculated from scratch using so-called lattice calculations running on a supercomputer. However, the result is far from the theoretical consensus value and has still to be confirmed by other independent calculations. But as mentioned earlier it already happened before that the tension between theory and experiment vanished after theorists reevaluated their model. When theory and experiment hone each other, they both become sharper.
So it is still unclear if the current Standard Model of particle physics has finally been cracked open. Many people doubt that the g-2 result is due to a new particle, because we should have already seen it in current particle colliders like the LHC. On the other hand, it could be just around the corner, to be found by current or near-future colliders. In the meantime, Fermilab is already busy analyzing some of their more recent data, and is still continuing data taking so we can expect a more accurate g-2 value soon. The topic is definitely one of the most exciting in particle physics right now and it is worth keeping an eye on it.
Brain hurts. Just give this man a soldering iron and some wires, or an IDE, something other than particle physics (not that I didn’t enjoy not understanding this article, though! :)
g = 2.001165±5 well that would be around 7 down to -3 then…. even I could replicate that in my barn!
Does this get us closer to practical Muon-catalyzed fusion?
Mooing Cattle what?
https://en.wikipedia.org/wiki/Muon-catalyzed_fusion
Who knows maybe we’ll have a maser at the resonant frequency or some sort of nano catalyst one day, to knock off a steady stream of muons and keep a desk top fusion reactor ticking over. Closer maybe but we still have a way to go yet before viable fusion is the public domain.
Very good article.
Well written and highly informative, with an easy, pleasant style on a largely-unfamiliar but highly important topic of physics.
Someone (I don’t remember who) said, “If I write to entertain, people will remember, and learn, and be impressed. If I write to impress, people will neither be entertained, nor learn, nor remember.”
Keep up the good, entertaining work.
May 18th Shenzhen