The power grid is a complicated beast, regardless of where you live. Power plants have to send energy to all of their clients at a constant frequency and voltage (regardless of the demand at any one time), and to do that they need a wide array of equipment. From transformers and voltage regulators to line reactors and capacitors, breakers and fuses, and solid-state and specialized mechanical relays, almost every branch of engineering can be found in the power grid. Of course, we shouldn’t leave out the most obvious part of the grid: the wires that actually form the grid itself.
The Difference Between Transmission Lines and Distribution Lines
There are typically two types of power lines that make up the grid, which can be divided based on their function. One group consists of smaller, lower-voltage lines (under 30 kV in most situations) which deliver power to homes and businesses. These are known as distribution lines, and can be buried underground in newer neighborhoods or are strung on smaller poles around 40 feet in height. The number of energy-carrying wires on them is three or fewer (per circuit, some distribution poles carry more than one three-phase circuit) and they tend to hold other equipment on them as well, such as transformers, fuses, switches, and even telephone and cable lines.
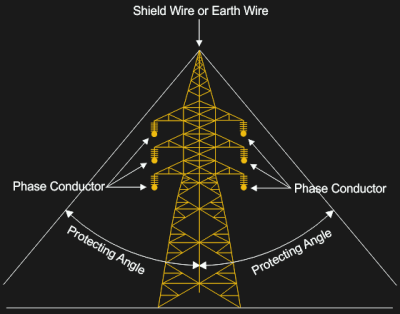
The other side of this division are the much larger, higher-voltage lines known as transmission lines. These can be easily distinguished from distribution by their larger size, but there are a few other indicators that you are looking at a transmission line rather than a distribution line. Transmission lines are always built with sets of three conductors with an optional small wire or two at the top of the structure to serve as lightning protection. While a typical residential service may only include a single phase, the electric grid itself is a three-phase system and the transmission lines are meticulously balanced so that an equal amount of current flows on each of the three phases.
Transmission structures also do not have any equipment on them that attaches to the power lines. A distribution line may have fuses, transformers, voltage regulators, capacitors, reclosers, or any number of other devices attached to the power lines themselves. Transmission lines will almost never have anything attached to the conductors themselves, although sometimes unrelated equipment are attached to the structures like cell towers.
Dealing with Incredible Voltage Levels
[Image Source: Electrotechnik]
This is where the voltages start to get a little bit out of hand. If you’ve noticed, so far I’ve referred to 10 kV as “low voltage” and 30 kV as “lower voltage”, each of which are well out of the reach of most engineers or hobbyists to handle safely. In any other world, these would be considered extremely high voltage. For the transmission lines, though, which handle bulk power, the voltages can be as high as 500 kV and still carry thousands of amps of current. This is necessary for moving power from a 4 gigawatt-capable nuclear plant, for example, tens or hundreds of miles to a population center. To get all that power moving around without causing major problems takes some special equipment, though.
Transmission Towers
Working from the bottom up, the first piece of equipment is the pole or tower that the circuits will be attached to. These can be from 50 to 100 feet tall or more (the world’s tallest is over 1200 feet tall in China) and as a result of that increased height can get expensive to produce. From a value perspective, it makes sense to balance strength of the structures with the total number of structures themselves. This economical approach tends to result in towers that can be spaced an eighth of a mile or less apart for circuits on the lower end of the voltage scale, 60-200 kV, and as much as a quarter mile apart for the higher voltage circuits like the 500 kV lines. Supporting a quarter mile of steel wire isn’t easy either, especially not if the circuit makes a turn, or if it traverses mountains or other obstacles.
To get the required amount of strength, some transmission lines are constructed on lattice towers. This is probably the most commonly used structure for routing transmission lines across the landscape, as it is relatively cheap to build them and they can be easily designed for varying heights and strengths as the situations demand. They can also be assembled at the final location, which makes it easy to get these structures to difficult-to-reach locations like isolated mountain valleys or sparsely populated deserts. There are some downsides, however. Lattice towers aren’t the strongest available structure in some situations, have a wide footprint which typically can’t be adapted for urban environments, and steel can be a very poor material choice in some situations, especially in coastal areas with salt spray or swampy areas with high humidity.
![steel-transmission-pole Steel transmission pole [Image Source: Bajaj Electricals Ltd.]](https://i0.wp.com/hackaday.com/wp-content/uploads/2019/06/steel-transmission-pole.jpg?w=430&h=242&ssl=1)
To compensate for the weaknesses of lattice towers, other structures are available. A popular choice when strength is a priority are poles made out of concrete and pre-tensioned steel rebar. Concrete poles have superior performance in hurricane-prone areas (and are surprisingly bendy), have less of a footprint than a lattice tower of similar height, and can be easier to install. The downside is that they are typically more expensive and must be built with specialized equipment and then trucked to the site whole. Steel poles can also be produced with similar performance characteristics of concrete, and some are even built out of a special alloy called weathering steel (sometimes referred to as corten steel, a trade name), which forms a protective rust layer only on the surface of the pole, protecting the structural steel underneath. Another advantage of steel is that it can be easier to produce structures with more than one pole (supporting the wires via a crossarm of some sort) for the largest of transmission lines.
High Voltage Insulators
Attached to the towers themselves are the wires, but in order to prevent massive and immediate faults the wires much be attached to the towers with an insulator. At these voltages, though, just a simple piece of glass or plastic won’t cut it, since the air itself will become ionized and form a path to ground for the electricity to flow. Specialized insulators are needed which can withstand the tremendous amount of electrical pressure placed on them. Before the modern polymer industry, long chains of glass “bells” were strung together and attached to the tower. These insulators were heavy, expensive, fragile, and required some time to assemble in the field. Now there are more advanced forms of insulators that are typically a single piece of plastic-rubber polymer that are both strong enough to withstand the electrical forces themselves, not to mention the extreme weight and tension of the power lines, and long enough to prevent the air around them from ionizing a complete electrical path to the tower. In fact, it is often possible to make a relatively accurate estimate on the voltage of the line based on the length of the insulators.
Very Strong Wires
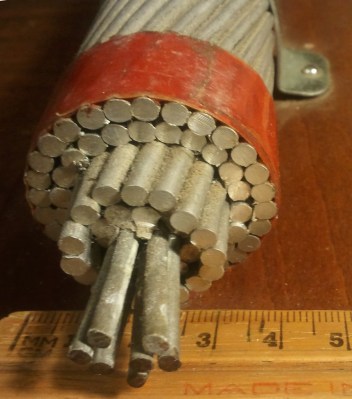
As one can imagine, the logistics of stringing the actual wires hundreds of miles on spans up to a quarter mile long can get a little interesting.
The tensile strength of most good and/or cost-effective conductors aren’t usually up to this task, so some interesting solutions were found to keep the costs and resistive losses down without stretching wires to their breaking point. Steel has no problem meeting these demands, but compared to other metals like aluminum or copper, steel is not a very efficient conductor. To get more out of the wires, some are built with a stranded steel core, which is then wrapped with outer layers of aluminum to improve its conductive ability. An interesting feature of alternating current is that the current tends to travel on the outside surface of the conductor rather than uniformly through the whole wire, which means that wires of mixed metals can get all of the strength of steel with almost all of the conductivity of solid aluminum.
Of course, different transmission lines will have different thicknesses based on the amount of current flowing through the lines. One major design consideration for these lines is how much they will “sag” under heavy load since the more current they carry the more they will heat up and expand, and the closer the wire will get to the ground. In some situations, overloading transmission lines has caused them to sag from the heat so much that they were able to fault to trees or other things in the transmission right-of-way and cause massive blackouts.
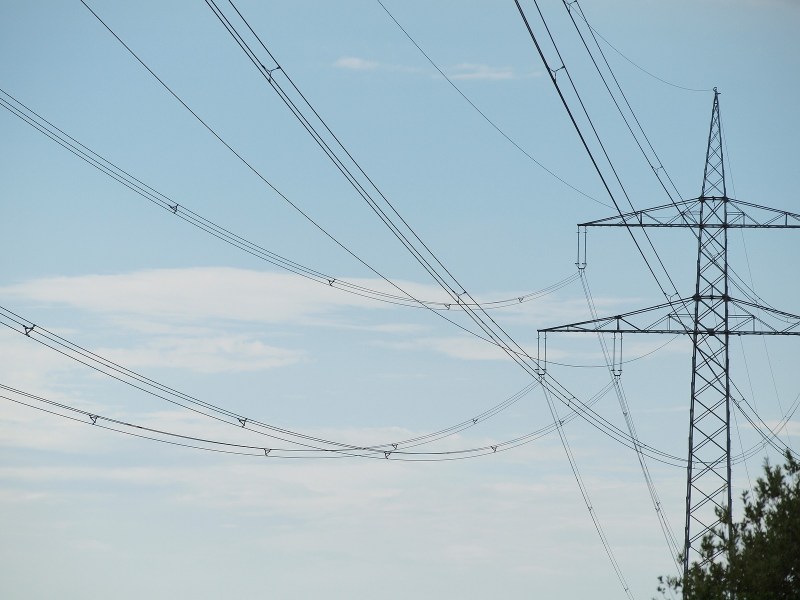
Thicker wires will heat up less for a given amount of current, increasing the carrying capacity of the circuit. One solution to increasing the effective thickness of a conductor is to “bundle” several conductors a few inches apart from one another, allowing for a larger increase in current for less cost than a conductor that is simply double the size.
The More Out-of-the-Ordinary Ways to Transmit Electricity
There are a few notable exceptions to the generic overview of transmission lines presented here. First of all, not all transmission lines are attached to towers or poles. Some are buried underground, although the cost of the specialized insulated conductors is orders of magnitude more expensive than overhead construction and therefore are only installed in places with extreme needs, like urban areas, under rivers or channels, or any place where it is cost prohibitive to build structures. Due to issues with the behavior of alternating current, it is almost impossible to construct a line longer than about 40 miles as well, leading to more design limitations with these types of circuits.
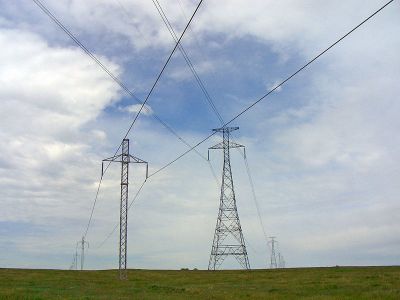
A second irregularity of transmission lines is high-voltage direct current circuits (HVDC). Due to the high cost of converting from AC to DC and back again, these lines are only constructed when power needs to be delivered extreme distances. DC lines do not come in sets of three conductors, but rather in sets of two. They are also immune from the charging losses that plague AC transmission lines, which allow for underground circuits of longer distances to be constructed as well.
Barriers to Improving the State of the Art
Moving into the future, it’s hard to say how much more modern the power grid can get since the underlying principles are so simple: three phases per circuit and structures large enough to keep them from sagging into something that could cause a fault. There is a lot of talk of the smart grid, but the solution to most of the issues with the power system is often simply to build more circuits as the demand for electricity rises. It’s a difficult problem to engineer ourselves out of, especially with the increasing age of the power grid itself, and at some point is simply becomes a numbers game of how many watts can be moved from place to place.
I feel so empowered know this :)
You’d enjoy http://amasci.com/amateur/whygnd.html too then.
Very nice read too indeed!
Cool article. Learned something. Thanks for the write up.
“Thicker wires will heat up less for a given amount of current, increasing the carrying capacity of the circuit. One solution to increasing the effective thickness of a conductor is to “bundle” several conductors a few inches apart from one another, allowing for a larger increase in current for less cost than a conductor that is simply double the size.”
Actually, cooling the wires isn’t the main reason for bundling wires.
Minimizing corona discharge is the main reason for bundling conductors, since the electric field isn’t as “sharp” around the wire. This is fairly crucial when dealing with very high voltages. (300 KV or higher typically is where one starts seeing bundled conductors. Though, some installers starts earlier for various reasons.)
Though, HVDC distribution is slowly replacing AC transmission lines. Who knows, maybe DC to the home is an option in a decade or two… After all, even fridges and other compressors are moving towards brushless DC motors due to much higher energy efficiency and lower material costs. And practically no other AC appliances exist in the average home these days…
A fair few datacenters already use “low voltage” (typically 250-450 volts) DC distribution in their server halls, due to power factor correction then not being needed in every server. But rather one big power factor corrector can be used instead when connecting to the grid. (Its is cheaper.)
Though, main advantages with DC over AC is that DC doesn’t exhibit the sink effect, nor does DC need to recharge the lines (twice) with every cycle. Then DC also doesn’t drop down to zero volts 100-120 times a second, so power supply regulation is more stable, though this very lack of crossing through zero volts 100-120 times a second also makes switching DC far harder when dealing with high voltages…. (Not that the mosfet in switch mode power supplies has all that much issue doing it tens of thousands of times a second.)
I can see a whole-house DC power supply and DC distribution in-home (perhaps as PoE) being a thing in the future, and certainly DC transmission is a thing today, but I still think distribution systems will continue to be AC certainly through my grandchildren’s lifetime. It’s just too big of a change to make, and making it gradually would be too chaotic.
A lot of electronic devices take advantage of properties of AC. The dropper capacitor is the best example, I don’t think there is a similar cheap efficient way to drop down DC voltage losslessly.
Yes, the capacitive dropper is a very simple power supply, can’t argue with that.
Though, “losslessly”, even a capacitive dropper has loss.
But in terms of LED lighting then a sufficiently long string of LEDs wouldn’t need much more than a current limiting resistor. Depending on what one’s DC mains voltage is.
In terms of other low powered devices, then it would likely not take long before some company makes a cheap, high voltage, single chip, buck converter that only needs an external inductor. (Actually thinking about it, I wouldn’t be surprised if such a device already exists….)
They absolutely do. I use them almost daily. Though in addition to the inductor you also need input and output capacitors, those can still be quite small.
Capacitors?!
Nah, who needs capacitors!
Okay yes, the input capacitor would greatly lower the amount of EMI our device spews out onto our DC mains. (and not make our supply collapse 100-120 times a second on AC.)
And okay, an output capacitor would help to reduce output ripple by a lot and make it useful for more then just a resistiv heater, LED lighting, and similar.
Sounds useful.
Do you have a link for these?
Answering myself here.
But I actually stumbled onto a single chip high voltage buck converter the other day when repairing an industrial sized hair dryer…
HVDC aren’t replacing AC lines in any real sense. HVDC lines are used for two big reasons.
1. Very long distance lines such as the one operated by Manitoba Hydro.
https://en.wikipedia.org/wiki/Nelson_River_DC_Transmission_System
2. HVDC lines are also used to transfer power between the various interconnections in Canada and the United States. When power is transferred from say the Eastern Interconnect and the Texas Interconnect (ERCOT). Using HVDC line means that the power can be transferred without coupling the frequency of the two grids. Essentially power can be transferred but the instability in one interconnect won’t effect the other.
https://en.wikipedia.org/wiki/Continental_U.S._power_transmission_grid
The ability to easily change the voltage using transformers in an AC system is essential. You can moving large amounts of power over long distances using high voltage transmission lines. Those high voltages would be an enormous hazard in a distribution setting though. AC allow us to reduce the voltage in the distribution grid that connects to our homes and businesses without using active components to change that voltage.
Imagine if the small distribution transformers that connect your home to the distribution grid were replaced by switching mode power supplies. The cost would be huge and losses would more than cancel out any other benefit.
“The ability to easily change the voltage using transformers in an AC system is essential.”
Yes, and in DC, we can use a flying cap inverter to do that with fairly high efficiency as well. When it comes to very high voltages. (Flying cap inverters becomes rather horrid at low voltages. (Horrid in power delivery, not efficiency.))
“Imagine if the small distribution transformers that connect your home to the distribution grid were replaced by switching mode power supplies. The cost would be huge and losses would more than cancel out any other benefit.”
Have you looked at the specifications of a traditional transformer? They aren’t 99.9% efficient, not even close to be fair. Most SMPS solutions are more efficient then a traditional transformer. (Mostly due to better core materials existing at higher operational frequencies. (Not to mention other design advantages…))
Then there is price. The main cost of an SMPS solution is the pure bill of materials cost. (Development isn’t that expensive, and its a one time cost…)
In the end, a switch mode power supply tends to have superior power efficiency (unless it has an absolutely horrid design.), and a far lower material cost then a traditional transformer.
Though, the main problem with DC to DC conversion at the current time is that cheap semiconductors gets hard to build above 5 KV ratings, and that greatly limits what voltages one can have at the input to the supply. (We could string many in series, but this comes with other disadvantages along with it.)
So until high voltage rated transistors without an insane price tag becomes reality, a DC grid is not economical at current. (This is though a greatly oversimplified answer, but hopefully it will suffice.)
“Imagine if the small distribution transformers that connect your home to the distribution grid were replaced by switching mode power supplies. The cost would be huge and losses would more than cancel out any other benefit.”
And….Harmonics. This I believe would be the biggest issue. It is already.
https://www.industry.usa.siemens.com/drives/us/en/electric-drives/ac-drives/Documents/DRV-WP-drive_harmonics_in_power_systems.pdf
Harmonics of what? The discussion on this comment thread is about HVDC transmission.
I don’t see how an article about typical problems with most cases where one sends AC straight through a bridge rectifier and into a capacitor without sufficient filtering nor any power factor correction, has any relevance to DC distribution on a grid.
Reason for why I don’t see the relevance is simply because, all the article talks about isn’t applicable if the grid were using DC instead of AC.
The only relevance this article has is mostly to point out the rapidly growing trend of using non linear loads. (computers, LED lighting, VFDs for motor control, industrial automation (PLC), and other similar systems.)
And since all these non linear loads has a bridge rectifier as one of the first things that the mains will see, then using DC distribution can have advantages. This is why more and more data centers are switching to DC distribution internally, then using one “giant” power factor corrector where it connects to the grid (typically many, for redundancy). And this is also not uncommon in larger industrial settings. (Though, some do power factor correction on the AC side of things. (as to not need to convert from AC to DC and back to AC again.))
@Alexander Wikstrom I am most familiar with tiny electronics, so this is not my field: How is a DC motor more efficient and cheaper than an AC motor? Before you answer I’m going to take a stab at my own question. From my limited understanding AC motors have been favored because there is less (or no) friction and I’m guessing new materials make the friction in DC less of an issue. I always thought DC wore out more often so lower material costs were not really the cheaper option. Although in fairness, even if im right in my guess the wearing out is more of a consumer problem than a manufacturer problem.
It’s not. You need to look up the way various AC and DC motors function. Careful, however, brushless DC motors are very much the same as a three phase AC motor, the rotation (commutation, switching the magnetic fields, so the motor spins) is created via an electronic motor control, rather than directly from the three phase power.
And even this leaves out the crucial difference: BLDC motors are really AC synchronous motors. All motors need some means of producing a phase difference between the stator and the rotor, so that there is a torque produced. Brush-commutated DC motors and AC/DC universal motors use carbon brushes and a segmented copper commutator to do this, and there are three undesirable consequences: friction, wear, and noise. Wear is the most significant consequence, because it means that brush-commutated motors need periodic maintenance. This was such a significant thing, the AC induction motor took over the industry in many applications. Induction motors produce the rotating magnetic field at the proper phase into the rotor by transformer action between the stator and the rotor, eliminating the brushes. But there’s a consequence to this as well – all of the current for the rotor has to go through the stator, making the stator larger and requiring more copper and iron, than an equivalent brush-commutated motor. But for many applications, the extension in life expectancy is well worth the extra weight and cost, which is why we see induction motors practically everywhere in stationary applications.
Brushless DC (and variable-frequency-driven AC) motors are a huge improvement over AC induction motors, because they eliminate the need for passing the rotor current through the stator, by using a rotating field in the stator to work against the field of a permanent magnet rotor, which of course rotates with the rotor. When first introduced, this was offset by the cost of the electronics required to produce the rotating field, so they didn’t immediately replace induction and brushed motors in all applications. But with improvements in electronics (particularly high-current MOSFETs and IGBTs) and microcontrollers, they are slowly taking over. These are making highly efficient refrigerators (“inverter” types) and electric vehicles, for example, possible.
A Brushless DC motor can ideally reach a higher energy efficiency then an AC motor. The AC motor’s main efficiency issue is that main frequencies are only around 50-60 Hz world wide.
Why this matters is because of simple things like energy loss in the magnet core, and how much energy the core is able to transfer with each cycle before reaching saturation. Not to mention resistive losses (both electrical and mechanical) and so forth…
In essence, a given electromagnet be it in a motor or part of a transformer, can only transfer X amount of power before reaching saturation. An electromagnet is technically an inductor.
The main thing with an electric motor is that we desire to push/pull the rotor around by alternating the polarity of our electromagnets. Typically by having 3 groups of magnets in a pattern like: ABCABCABCABC (this would be a 4 pole motor, but this is unrelated.) These 3 groups are then have a 120 degree phase offset from each other. Thereby forcing the rotor to spin in our desired direction.
But then comes the question, why would a brushless DC motor be more energy efficient then most AC motors?
The reason is similar to why Switch mode power supplies are more energy efficient compared to a traditional transformer, despite containing a transformer at its heart.
It all comes down to quickly ramping currents as to induce a strong magnetic field in the core material, instead of burning it restively, and then ramping the current back through zero and starting sending current through in the other direction as to revers the magnetic field. (This is though a really crude explanation and highly oversimplified.)
Though, realistically we will always burn a portion of the energy restively due to wire resistance alone, while the magnetic core will add its own impedance on top, this though reduces as the inductor/magnet builds its magnetic field. In other words, the slower we alternate the polarity, the more resistive losses we will have.
So going for higher frequencies is going to reduce resistive losses, then there is core losses, mechanical losses ect… Downside with higher frequencies is that for a motor with a given amount of poles, then its RPM would equal the operational frequency divided by the pole count then divided by 60, unless one likes RPS. This means, higher frequencies usually also comes with higher RPMs. This can though easily be fixed by either using more poles, or some gear reduction on the output.
An advantage with higher operational frequencies is, that a given magnetic core can only transfer a fixed amount of energy per cycle before reaching saturation. This means that with more cycles per unit of time, we get more total energy transferred. Thereby increasing our energy density. And it is here that we could then start cutting down the physical size of the motor and make the material costs for it cheaper. (Ideally that is, but last I checked, large industrial brushless DC motors were a bit of a luxury compared to AC ones…) Also, a smaller size saves on weight, this is why airplanes use 400 Hz AC distribution instead.
Another advantage with brushless DC motors is the pure fact that they typically are electronically driven, so that one can have it run at any desired RPM (within specifications), and do soft starts, acceleration, de-jerking, etc.
Though, then there is brushed motors, and that is another can of worms altogether… (usually more then noticeably less efficient, wears out over time, etc….)
But I should probably end on the note that a brushless DC motor is technically just a 3 phase AC motor with a fancy controller and optimized for higher frequency operation.
Much of what you have said is incorrect. Brushess DC motors are AC syncronous motors with electronic conversion DC to AC. More cycles per secon will not increase energy transfer- in fact it will result in higher core losses. Magnetic cores are sized to operate below the knee of the saturation curve- this is also true for transformers. Aircraft use 400 hertz to save weight and size but airplanes are small and 400Hertz transmission for longer distances is not feasible. Transformers can be made to be in the 99.9 % range (large ones are there) but to do this means increased costs.
Lower frequencies do not necessarily increase
What do you mean by electric field isn’t as ‘sharp’ around the wire? What do you mean by ‘sharp’?
Corona reduction is the original objective of using bundled conductors as an approximation to a large diameter cylinder ( the original conductors at Boulder Dam were hollow copper abut an inch in diameter with about 1/8 inch wall thickness. ). There is some economic and mechanical gain and,mainly, reduced inductance. (with an associated increase in capacitance ). The surge impedance is lower as well . The highest voltage substation I have been in ws 750KV and an adjacent lav was testing 1200KV structures.
HVDC is good for longer distances or where underground or underwater cables are involved. They alos can connect ac systems with back to back converters to avoid synchronizing an stability problems.
Brushless DC gains some energy efficiency with permanent magnets for excitation- but are basically AC “synchronous” machines with electronic switching so the efficiency gain is not due to being “DC” but is due to the improvements in magnets and switching.
In the “North Country”, during a cold snap, there is very little sag between power poles.
Cold plus rain gives ice coated wires, which ‘sag’ right to the ground, along with crumpled towers.
I’ve always thought that when those conditions were immenant, the utilities should reduce the voltage and increase the current. The resulting increased heating might prevent or reduce the icing, saving the lines from destruction.
AFAIK, some of the lessons and methods developed after the 1998 ice storm include something called the ‘Levis de-icer’. It injects high-voltage DC on top of the 735kV AC of the long-distance transmission lines to melt the ice. Since there’s no change to the AC voltage it means the substation transformers don’t need to be adjusted on-the-fly.
Since the de-icing function isn’t needed all that frequently it also doubles as a static VAR compensator.
https://en.wikipedia.org/wiki/Levis_De-Icer
Hydro Quebec also came up with new HV transmission tower designs that can handle a much higher weight margin than the old towers did, while using less metal.
Wow! another use for my old Levi’s jeans!
B^)
utilities cannot do that. The utility provides a voltage and, the load determines the current. The combination is the power then produced and delivered. In the simple case of a lightbulb, a lower voltage will result in a lower current and less light. With a motor there would be poor starting or poor operation, possibly stalling under load. What utilities can do in some cases is it circulate current around a loop to provide some heating.
See these weird-looking tripod transmission line towers crossing the Mississippi in Minneapolis, MN
https://photos.google.com/album/AF1QipPQnSYlp8ecw0evHkcbmht4_VuYYmIFHvSrs1Wb/photo/AF1QipND6OY3ig2yxCced0cXuy5pf-4Gh1zALPv_18O4 — there’s three of them from left to right.
Whoops, try this link instead
https://photos.app.goo.gl/snLdCGbezMp7cCwr7
Those are unique. There are many odd towers around – bet you can guess where this 230kv tower is…
https://en.wikipedia.org/wiki/Mickey_Pylon
Those are architectural structures. They were built to not be as much of an eye sore in the area of the historic Stone Arch bridge.
Awesome detailed article. Thanks for posting
My grandfather was an electrical engineer in the Southwest UK in the ’60s and ’70s.
He helped with the design of substations, and I believe his main job was to ensure the overhead lines were clear before the juice was turned back on after maintenance. Thankfully, no-one got fried on his watch.
He had several anecdotes about the ‘old guard’ way of working, compared with the ‘fresh out of technical college’ youngsters.
Old: 1000 yard drum of cable, 600 yards for job 1,
360 yards for job 2, 40 yards or so scrap.
New: 750 yards for job, rest scrapped.
A power line was to be routed down a valley. PFY plotted ‘best route’ , which was quite economical, except for the part where it went *through* a church tower…
https://goo.gl/maps/xEMhgth5VHz4b3hF8
I don’t know if the fuel pump was built after or before the transmission line. I suppose they found the hard way that induced currents and petrol don’t match.
https://goo.gl/maps/osFfeoU6esuqQbcR6
The twin fuel pump in the other side of the road is more successful (ok, maybe it’s not for the scary wires, but because it’s before the motorway entrance)
Boy I can tell you there is nothing like having to work inside of some of the largest Transformers in the world.
I have been a electrician for over twenty-five years.
If you have ever had been in a tank. Its like that but even tighter.
It was all kind of yellow-orange inside. Lots of bare copper, paper covered copper, big red insulators and lastly a lot of steel. Everything is big (very Big).
One thing I didn’t know was that to get any large transformer ready, you have to dry it all out. So that there is no moister in side. This can take anywhere from 1 week to all most 2 months in the winter.
Then they fill it up with oil. If my memory is right it took about 20 50 gal drums of oil for a 500 000 volt Transformer.
The type of work I have done has been so so cool.
I really loved my work.
Very good posting.
Thanks for the flashback.
One more little point. I saw this when I was in the Army.
We were on a exercise in the mountains of BC Canada.
I was on post and on the other side of the valley were Hydro lines.
After your eyes got used to the dark. You could see the hydroline glow green.
It was soo cool.
St. Elmo’s Fire?
Airbus over Argentina experiences rare St. Elmo’s Fire effect >>>
https://www.reddit.com/r/woahdude/comments/7x0u0n/airbus_over_argentina_experiences_rare_st_elmos/
They used to have a transformer here in Seattle WA north of Georgetown that was two stories tall about 50 feet across. The two building around it and the chain link fence had to be electrically grounded. I never saw something that big before. Even the transformers that feed the area around the Space Needle are not that big. This was an old one. I could not find a picture of it now. They removed it.
Local generation – in particular with smaller, next-gen nuclear plants and smaller solar and wind installations is the way forward and in principle can reduce the need to move power around long distances (and reduce carbon emissions).
No, it won
No, it won’t reduce carbon emissions, and it’s not the way forward. Local generation makes sense on remote areas, where the cost of transmission lines offset the cost of building smaller plants, but for the vast majority of the planet population, a centralized large plant makes more economical sense.
Local generation means more waste. There will be maintenance for lots and lots of smaller plants, and it’s way more costly to fix lots of small pieces than a larger one. And decomissioning will be a nightmare too. Monitoring and power management on a cluster of small plants is way more complicated than a few beefy plants. Imagine plugging thousands of small plants on the grid and defining how much power to draw from each one, in real time.
But for a small minority, makes sense. People on the White North, on tropical forests, deserts. In this limited cases, a small local power plant is more viable.
Managing power of on site wind and solar is easily done by using the power on site. Thermal storage is very economical for HVAC and hot water, which comprise the majority of residential energy use in most homes.
What you guys are beating around the bush (a lot) about is Distributed Generation. A great idea that everyone agrees is the way forward; unfortunately no one can figure out how to do it economically. Safety and C&C (command and control from the local grid’s control room) are major thorns. There’s also the major political issues where the power companies are fighting tooth and nail against it because it will reduce generation revenues.
“the voltages can be as high as 500 kV”
actually the highest voltage lines are slightly north of 1MV
The AEP system uses 765 kV lines extensively in Indiana (and other parts of their system). My husband used his forestry degree (the part that knew how to read maps and land description records) to contact landowners across southern Indiana to negotiate line rights-of-way for one that size. At one time AEP had a section of 1 MV line to do R&D, I haven’t heard if they ever moved that size into production.
Some days I add to my Google map of power distribution in the SE UK.
https://drive.google.com/open?id=1vxyUTxBihFdHW1foby4m7vnzaVRtJynH&usp=sharing
I recently became interested in how the substations were inerconnected and I started to plot out the high voltage lines on a google map as well…. waiting now for a black car to pull up and take me away :-)
Same here.
Take a look at contributing to open street map, or checking there for existing lines.
https://www.openstreetmap.org/#map=16/50.8382/-0.2226
Pretty neat.
For those with an appetite for even more excitement, “Enter, Stage Center: The Early Drama of the Hyperbolic Functions” is an interesting read about catenary curves
https://www.maa.org/sites/default/files/321922717729.pdf.bannered.pdf
Transmission wire temp ~!00degC. Newer lines are up to ~200degC.
I’ve always wondered about the type of tower where one conductor goes *though* a hole in the center of the tower*.
These towers are common where I live, and I’ve always wondered why the tower structure, which seemingly forms a continuous ring around the conductor, does not act like a closed secondary transformer winding, inducing a bunch of current in itself.
I’ve looked at a few towers over the years and I’ve never been able to see an insulator breaking the ring.
The geometry isn’t perfect for a transformer, of course, but there’s enough structure in parallel with the wire and the fields are high enough, that I would expect a good bit of coupling. I would think that even if a single tower could be ignored, the cumulative effect of a string of towers would unbalance the phases.
*see the illustration at the top of the article for what I’m talking about
Story has it that an engineer working on transmission lines one day started looking at gas station sign towers, and wondered how those stay standing. Turned out, that same construction method works for transmission lines as well.
As to why those don’t act as windings… I don’t know really, but my guess is that it’s because all three phases pass through the tower, and between them there’s no net magnetic field that could couple to the tower.
ha! power would only be induced into the tower when there’s a ground fault somewhere else (think GFCI).
Ad a light in that ring and you can literally see where the ground fault is (between which towers).
Unless of course the complete transmission line is isolated from ground (so no voltage potential between any of the phases and ground – only between the phases).
The reason that these towers don’t act as windings is that you need a lot of windings to make a noticeable impact on the current flowing through any conductor, and this type of tower is essentially just a single winding, of which negligible current is induced. For something like a current transformer, which takes advantage of inductive coupling, it may have thousands of windings in a comparable amount of space. Even for inductive coupling of a transmission line to things like fences running alongside power lines, there are typically not problems unless the fence is ~50 miles long. It takes a lot to make a difference with this effect.
Yes , there is a magnetic field around the conductor but it would produce a voltage (transformer effect in a conductor parallel to the line-
Or, it could go into personally held power generators instead of a pricey grid.
You mean y’all aren’t using Zero Point Energy modules to power your house? What century are you living in ?
At only about ten times the cost per KWH.
One item on the high voltage transmission lines that was not noted were those little “barbell” looking attachments to the conductors at esch end of a span. These are called Stockbridge dampeners and their purpose is to minimize aeolian vibrations in the conductor that is typically caused by the wind. Without these the conductor could ultimatery fail because of the vibrations. A quite nice article
Also not mentioned: corona rings around the ends of insulators, which reduce the electric field gradient at those points. Used almost everywhere bundled conductors are.
Got on just to post roughly the same info. I deal with fairly high voltages (for a hobbyist) of say 50kv DC + 30kv RF (a fusion hobby setup). At some point, the air alone won’t divide a drop between your supply and the nearest ground evenly. Once you get a corona streamer started (maybe a little ionization from a cosmic ray?) – it’s pointy, concentrates field around the tip and extends – often without obvious length limit. Electricity under these conditions does NOT always take the shortest path. I’ve had an arc at 100kv – on a foot long vacuum feedthrough – that jumped 6 feet almost to a grounded milling machine, made a U turn, and landed back on the opposite side of the vacuum tank it began on. You need new underwear if that happens.
Look at lightning. Ever seen it straight? Nope, a streamer just “follows its nose” along little ionization tracks, specks of dust and so on (which cause gradients in voltage due to their dielectric constant and the not quite DC nature of all this), and once a continuous track if set up, the main bolt happens.
Without that, there’s not enough voltage available to make it the length of the stroke!
When you get up to these really huge voltages, and in the DC particle accelerators I’m more familiar with – you use corona rings to force a gradient that’s evenly divided to prevent arcs – you really need that little bit of evenly spaced losses (like a bunch of resistors in a divider) to make this all work.
So what electricity sees as the shortest path isn’t shortest on a map, necessarily. And that’s ignoring Paschen’s law, which means at lower pressures in gas, it might have to go a longer way to “hit” enough atoms to maintain ionization (ionized gasses are far more conductive than neutral – else your various arc welders wouldn’t be able to pull nearly inch arcs with 30 volts…). I’ve had in-tank arcs of 2′ when the nearest path to ground was under an inch.
Yyyyup. What was a real eye-opener for me was seeing lightning in ultra-slow motion. You can see the stepped leaders just making ionized trails to nowhere-in-particular – just connecting random pockets of charge – and then one of those steps happens to make contact with something other than air and raindrops, and suddenly what may have been a few amps is tens of thousands.
Or, look at the arcs that form when a high-voltage (300 KV or higher) AC circuit is broken. The current follows the ionized airflow, and the airflow is pretty chaotic, since the air is being heated several thousand degrees in a few milliseconds.
Sounds like you’ve had more fun with this than I ever care to.
Principle of Least Action.
Thanks! I’ve wondered about those things on and off for around 30 years!
Transmission line “galloping” can be a big problem where the lines are subjected to high winds.
The article could have also linked to Dave Barry’s explanation of electricity, which would have helped a number of HaD readers in understanding how electricity works.
http://www2.hawaii.edu/~darlene4/dmed120/assignment12.htm
There’s quite a nice summary of the many flavours of stockbridge dampener at http://www.dulhunty.com/an1.htm
Why are transmission lines that high above the ground?
I got to wondering this while driving across Columbia river basin – lots of open space, and transmission lines. Wide open land, lines are way higher than any trees or structures, lots higher than any snow could get.
I assume there are engineering or such factors that dictate the height of the lines. What are they?
Yes. There are induced currents in the ground. The further away from a three-phase transmission line you get, the more the electric fields from each conductor cancel each other out.
‘Induced Currents’ – There’s a disc golf course under some ‘lowish’ transmission lines near me – they induce enough current in the metal goal baskets that touching them has a decent potential. With sandals on- you are insulated from the ground enough that the grass blades touching your skin around them completing the ground path from the basket feel like they’re burning your skin. Never thought too much about it, but the basket must have a concrete footing insulating the post/basket for the induced potential to want to go through a body…
The conductors are high to keep the near ground l electric fields at a safe level, there are regulations which deal with minimum level stadards.
Insulator question….
Way back in the college days the reason for the curvy ‘stacked dishes’ shape of the insulators was explained to us. Now it’s long gone from the gray matter.
Anybody care to explain?
Thanks,
Queeg
Two reasons. First, for any dirt and contamination on the insulator, the resistance is proportional to the path length, and the path length is greatly increased by the zig-zag shape. Second, as rain washes said contamination off the insulators, this helps in one of two ways: a) for vertical stacks, the water flow is broken into drops at the edges of the discs (the discs there are bowl-shaped, so water won’t flow back toward the axis of the stack, so there’s no continuous liquid flow path, and b) for horizontal or angled stacks, water drips off the edges into free air, again preventing formation of a continuous stream of water from one end of the insulator to the other.
Thanks!!!!!!!
The skin effect at 60 Hz? You would not also be selling super conducting oxygen free pure gold speaker cables to the audio nuts would you?
At 60 Hz, skin depth is 8-9mm; many transmission lines are larger than that.
Well, yeah, skin effect. See, those cables have a twist, right? So the electrons get slung to the outside by centrifugal force, which forces them to the top, causing a skin effect. Simple physics, right? :)
You find this exciting? Well, well you just sit right down then! I’ve got a slide show of particularly interesting 20th century telegraph poles I’m sure you’ll enjoy!
I’ll warm up the old Hammond while we watch!
And do let me know if you feel the urge for some Morris dancing!
“Moving into the future, it’s hard to say how much more modern the power grid can get since the underlying principles are so simple”
At some point, room temperature superconductors, we hope.
If I ever get my hands on the idiot who decided that these transmission lines should get the same name as constant-impedance RF transmission lines, …
While you’re at it…you could add the idiot who did this…
https://en.wikipedia.org/wiki/Transmission_line_loudspeaker
While you’re at it, you could add this idiot…
https://en.wikipedia.org/wiki/Transmission_line_loudspeaker
All of these things are properly called transmission lines. The same principles have to be followed. And HV transmission lines ARE constant impedance. The only difference is the frequency.
NO, just NO. In EVERY world Low voltage is ONLY ever 1000Vac or less. No Electrician, Linesman, Engineer or anyone in the industry, under any circumstances, would EVER refer to anything over this as Low Voltage, and for very good reasons.
It is not only wrong but idiotic, ignorant and extremely dangerous to confuse or miss use these labels.
“so far I’ve referred to 10 kV as “low voltage” and 30 kV as “lower voltage”
So not only are you dangerously mislabeling very well established Voltage classes, now somehow “lower” refers to something higher than “Low”, only in your your world.
I’ve heard some RF engineers say that anything less than 1GHz is DC.
Anything less than blue is red.
If you’re interested in checking some EMF in transmission lines, I’ve got an example with calculations and simulations that I did as a project at the university: http://thefragmentationparadox.blogspot.com/2014/03/electromagnetic-fields-emf-in-high_16.html
“”it is almost impossible to construct a line longer than about 40 miles as well, leading to more design limitations with these types of circuits.””
I don’t think this is correct. Why should AC lines be limited to 40 miles?
It takes light 0.000215~ seconds to travel 40 miles,
The inverse of 120 Hz is 0.00833~ seconds, (the number times/second that zero crossover occurs)
0.000215 is close to 1/40th of 0.008333…
But then the speed of electricity in a cable is less than the speed of light,
Now, derive that from the number of pancakes it takes to shingle a doghouse.
Is that an African or European doghouse?
Here is a better picture of the rout of power lines between Arizona and southern California >>
https://mikediamondservices.com/blog/los-angeles-electrical-power-supply/
factor of 1000 error here?
At 60 hz a wavelength is 5000 km. 3125 miles
This 40 mile limitation is only true of underground transmission lines where all three phases are contained within a single cable with a diameter of about a quarter meter. At 230 kV, for example, the dielectric material of these cables is withstanding a voltage of ~2,000,000 volts per meter. The amount of energy needed to charge each phase every cycle of the AC waveform at these gradients increases as a function of distance, as more and more copper needs to “charge” before useful energy can be conducted through it. At 40 miles, the lines start using up an uneconomical amount of energy just to charge and discharge every cycle. At some distance, they would be using all the energy they could carry just for charging. This occurs in overhead transmission lines as well, but since the voltage gradient is less severe, they can be built longer. You do see charging losses on these start to exceed economic limits, which is when electricity providers start to build HVDC. The proper name for this effect is “charging loss”.
Thank you for this explanation.
That sentence referred specifically to undeground lines, which have a lot higher capacitance per unit length than overhead wires. There is a lot of good information on the reasons why in this link: https://www.ee.co.za/article/effects-of-mv-and-hv-cable-capacitance-on-operational-limits.html
On top of this, ground (and especially salty sea water) is conductive, resulting in eddy currents being created and causing thermal loss.
This is why all the underwater lines in Japan, Europe, etc are all HVDC: usually not at “high” voltage by HVDC standards (because of the insulation) but because these losses will not occur.
I was thinking the same thing. The Line between Los Angeles California and Arizona got to be more than 40 miles. >> http://vielmetti.typepad.com/.a/6a00d8341c4f1a53ef01543547fde5970c-500wi
Again, I don’t know where you got the idea it’s underground because it’s not: it’s a 500kV line running from Palo Verde Nuclear Generating Station to somewhere over there. It is a normal 3 phase overhead line. Even the lines running from PVNG to here in Phoenix 30 miles away is 3 sets of 3-conductor-per-phase 500 kV line to the major substation(s). (We have the 3rd largest capacity grid here in the USA: may have something to do with all those air conditioners, data centers, and semiconductor fabs! :)
that refers to underground lines which have high capacitance. a 30 mile cable is roughly equivalent to a 300mile overhead line
“Moving into the future, it’s hard to say how much more modern the power grid can get since the underlying principles are so simple:”
Modernizing the power grid means modernizing the parts of power grid that most people never see. The transmission system is protected from faults using a system of protective relays. Voltage transformers and current transformers are used to reduce transmission voltages and currents to a level that is safe to measure. These voltage and current signals are then fed into various types of relays that monitor the system for fault conditions. These relays are designated by their function using ANSI device numbers.
https://en.wikipedia.org/wiki/ANSI_device_numbers
A good example is a “21” distance or impedance relay that uses voltage and current to measure the impedance of a transmission line. Simply measuring for high current on a transmission line isn’t enough. A fault with low impedance might produce an abnormally large current but what about a high impedance fault? The high impedance fault might mean that the line is laying on the ground where it would be a huge hazard but the current on the line is still relatively normal. A distance relay uses both the current and voltage on the line along with expected impedance to detect the presence of a fault. On newer relays the location of the fault can be expressed in miles from the substation, making finding the fault much easier.
https://en.wikipedia.org/wiki/Protective_relay
When a fault is detected the relay send a signal to the breakers on either end of the transmission line to open and interrupt the fault. In some cases, the breakers might automatically reclose after a time delay to restore the line if the fault was only momentary.
In the past, the relays providing the different protective functions would be discrete elements. A transmission element such as a large transformer might have relays for over-current (50), differential current (87), sudden over-pressure (63), high temperature (23) conditions. For a fault, these relays would send their signals to another relay (52) responsible for actually opening the breakers and interruption the fault. A breaker failure relay (50BF) would then check to see that the required breaker had opened as expected, if not the next breaker down the line would open as isolate the fault. On top of this, a lock-out (86) relay would actuate to prevent re-energizing the faulted equipment.
For the transmission systems operators in a control center the actuation of these relays and subsequent breaker operation would appear as a general alarm at the substation where the relays and breaker operated and the change in status on their SCADA screen or map board for the breakers involved. They would then have to dispatch a technician to the substation were the fault occurred. The substation could be dozens or even hundreds of miles away. The technician would then have to inspect the relays looking for dropped “flags” to tell them which relays had actuated and why.
Modernizing the grid in many cases means replacing these discrete electro-mechcanical relays with a single solid-state relay capable of performing multiple functions in one package with the ability to communicate more information back to the control center. In the case of a powerful thunderstorm covering a large geographic area this information helps the transmission system operators stay informed and able to manage resources best. They can make better decisions sooner, use resources more efficiently, and keep the system as intact as possible.
Smart Meters.
there are also the big don’t-try-to-land-here balls you see on the lines where they cross possible emergency landing spots.
I do wonder, though, about the cell phone gear i often see mounted on the towers. It seems like the worse possible place to put a cell……… except for say, a tank of heavy water.
Top-hat cellular equipment works great, and avoids tower construction hassles with the local NIMBY brigade. 60Hz is literally DC as far as the RF equipment is concerned, there’s no interference as long as the AC connections are properly made and not sparking or exhibiting corona discharge.
It’s a bit weird working on those sites, you don’t get to just scamper up the tower and adjust downtilt or something, but the stuff at the base is just bog-standard radios and such. And often there’s buried fiber running in the right-of-way that’s already been cleared of brush for the tower route, so backhaul is trivial.
Misuse and abuse of the word “literally”.
Except that “literally” literally also means “figuratively” these days.
https://www.merriam-webster.com/dictionary/literally
And that’s double-plus-good by me!
That’s like saying “There are a lot of child molesters so child molestation is normal.”
One reason is they have to be higher than you might expect is to maintain clearance for the lines to safely sag under their maximum load.
I live in on the shores of Cahora bassa dam in Mozambique and we have a DC line that connects to South Africa that is well over a 1000 miles. There are 2 lines with earth return operating at 533 kV plus and minus spaced apart about a km . Polarity of the lines are swopped periodically to handle corrosion issues .
It makes sense that transmission towers are essentially designed to just get electricity from one place to another without problems. I imagine that it could be pretty complicated taking care of these due to the nature of the high voltage of electricity present though. Using trained professionals would probably be your very best option for keeping people safe when working on these.
Hey, very informative mate. I’m in Australia and as an operations manager for a large vegetation services company clearing transmission easements for TransGrid around NSW found this very interesting. I knew the basics of how it all works, and am very familiar with the sag and sway under loads ( extreme/ normal identified by LiDAR ) but it’s good to fill in the blanks, of sorts.
I may now pass this information onto customers who are always complaining about trimming or removing their trees within the easement when on their property, or generally asking why we do what we do.
Thanks
I am not an electrican or electrical engineer, but I have a question about how lines are chosen. Do residential water wells pose a problem if they are too close to transmission lines and/or distribution towers? I live in an area that is growing and they are building a substation down the road and want to run lines behind my neighbors homes. But when I looked at the constraint map that the energy company put out, they don’t show all the water wells. They only have numbers about 1% of the wells that are actually close the ROW and within area that will be directly affected. Does that matter and if so, how?
Thank you so much for your help!
in normal operation these lines will not have any effect as they will not cause ground currents .Even during faults which would be very short intervals, the ground currents would be parallel to the line.
A partir de que tensão elétrica de transmissão , e de que ´´indice cerâunico (número de dias /ano em que se ouve trovejar), é recomendado o cabo de terra em linhas elétricas aéreas de transmissão de energia, e quais as expressões que deverão ser utilizadas para o calculo da secção desse cabo de terra.
Aguardo resposta à questão formulada
The intricate nature of the power grid reveals a fascinating blend of engineering disciplines, from transformers and voltage regulators to the very wires forming the grid. Understanding the distinction between transmission and distribution lines clarifies their unique roles: distribution lines deliver power locally, while transmission lines handle high-voltage, long-distance power transport. Transmission lines, built with sets of three conductors and often supported by lattice towers or steel poles, require robust insulators and special wire constructions like ACSR to manage the immense voltage and current. Innovations such as HVDC lines and underground cables address specific challenges, but upgrading the grid remains complex. The push towards a smart grid highlights the ongoing need for modernization, balancing efficiency, sustainability, and reliability in meeting growing energy demands.
This is an “AI” generated comment on this article from a Chinese tower manufacturer
But… Why?