For decades scientists have been building detectors deep underground to search for dark matter. Now one of these experiments, the XENON1T detector, has found an unexpected signal in their data. Although the signal does not stem from dark matter it may still revolutionize physics.
Since the 1980s the majority of scientists believe that the most likely explanation for the missing mass problem is some yet undiscovered Weakly Interacting Massive Particle (WIMP). They also figured that if you build a large and sensitive enough detector we should be able to catch these particles which are constantly streaming through Earth. So since the early 1990s, we have been putting detectors made from ultrapure materials in tunnels and mines where they are shielded from cosmic radiation and natural radioactivity.
Over the decades these detectors have increased their sensitivity by a factor of about 10 million due to ever more sophisticated techniques of shielding and discriminating against before mentioned backgrounds. So far they haven’t found dark matter, but that doesn’t mean the high-end sensing installations will go unused.
No Dark Matter So Far
Unfortunately, apart from a long-standing unconvincing claim, WIMP searches have yet found nada. Still, the search continues and everybody hopes that WIMPs are just around the corner as experiments will soon hit the sensitivity limit due to the background from neutrinos. Lately, people have developed ambiguous opinions of whether the field is already dead and some argue that we should diversify the search for dark matter instead of focussing on WIMPs.
The dwindling hopes of detecting WIMPs also inspired the collaborations running these experiments to think of other interesting physics searches. After all, having a super-sensitive detector not only lets you search for WIMPs but also for other exotic particles and rare nuclear decays plus one can do some interesting neutrino physics. Having a broad physics program helps to secure funding and keeps your Ph.D. students busy. Earlier this year the world’s most sensitive dark matter experiment, XENON1T, was able to measure the double electron capture decay in Xe-124. With a half-life of ~1022 years this is the rarest decay ever measured, 12 orders of magnitude longer than the age of the universe.
Detecting Particles in Liquid Xenon
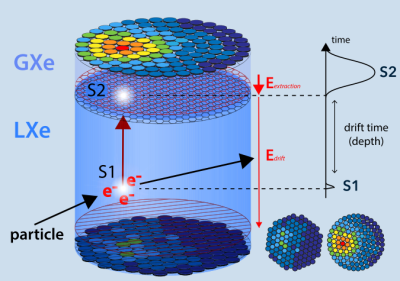
Credit: E. Shockley
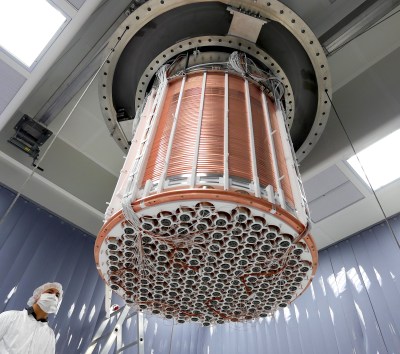
Credit: XENON1T collaboration
The XENON1T detector is a so-called dual-phase time projection chamber (TPC). It consists of a cylindrical tank filled with the noble gas xenon cooled down to its liquid state. Above the liquid, there is also a gaseous phase, and an electric field is applied between the two. Whenever a particle interacts in the liquid it produces a flash of light (S1) which is detected by an array of light sensors located at the top and bottom of the tank. In addition, the charges produced in the particle interaction are swept to the top by the electric field and produce another flash of light (S2) when they enter the gas layer. From the time difference between both signals S1 and S2, it is possible to reconstruct the z-position (height) of the particle interaction while the distribution of light seen by the top and bottom arrays allows the array to determine the xy-position. In addition, the energy deposited by the particle can be deduced from the combined intensity of both light flashes while their ratio S2/S1 provides some information about the type of interacting particle. The latter makes it possible to distinguish if a particle interacted with the electrons of a xenon atom, as is the case for radioactivity from beta decays and gamma-rays, or if the particle bounced off a xenon nucleus, as WIMPs or neutrons do.
An Unexpected Signal
While an earlier published WIMP search did unfortunately not reveal any signal, in their latest analysis, the XENON1T collaboration looked more closely at the electron recoil data which is normally disregarded as background. As mentioned, most of these types of events come from radioactive contamination of the xenon and the surrounding materials. This background was modeled by Monte-Carlo simulations and constraint by separate measurements, e.g. by the previous screening of materials for their radioactivity. When the collaboration looked at the data and compared it to the background model this is what they saw.
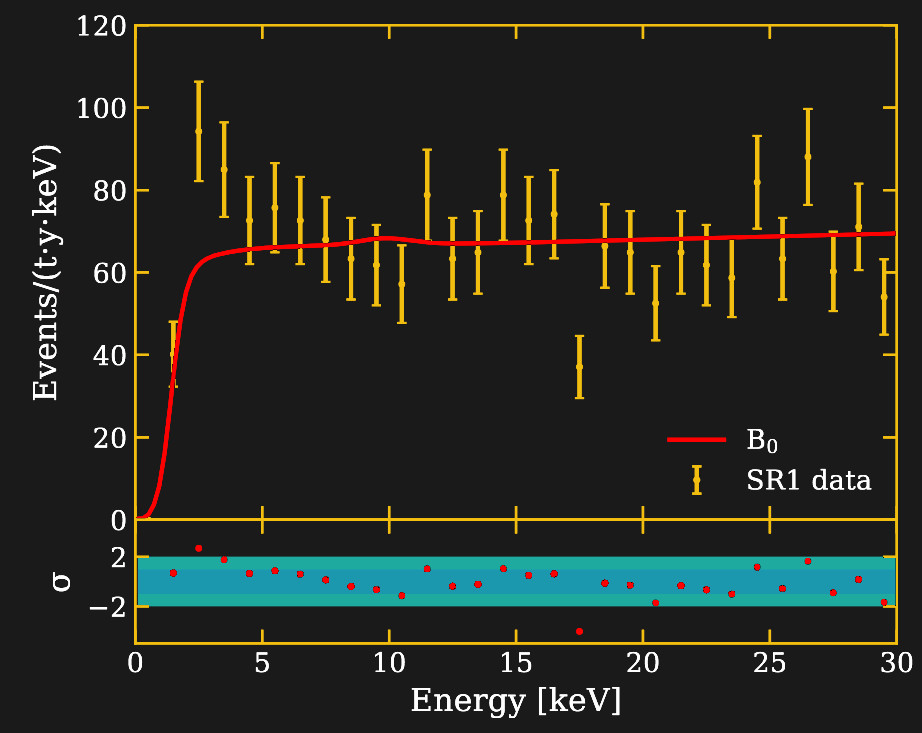
The data shows an excess of events between energies of 1 to 7 keV which peaks around 2 keV. By counting the events in this energy region and comparing it to the number of expected events from the background model, one gets a 3.5-sigma fluctuation. This means the probability to observe that many events for the given background model is merely 0.02%. While this sounds like it is safe to exclude a statistical fluctuation there have actually been a number of excesses in particle physics with a similar significance which later vanished. The most prominent example is the 750 GeV diphoton excess at the LHC, which had a significance of up to 3.9 sigma and led to the authorship of over 500 articles but turned out to be just a statistical fluke. Still, the XENON1T result calls for some interpretation of the data, and the collaboration studied three possibilities.
Did Someone Drop Their Watch?
According to Occam’s razor the most likely but also most boring explanation would be some kind of yet unaccounted background. It turns out that the beta decay of tritium, the unstable form of hydrogen with two additional neutrons, has a shape that resembles the observed excess. Tritium is also the stuff that excites the phosphor in self-glowing watches. A tiny fraction of hydrogen atoms, about 10-18, are tritium which is naturally produced by cosmic rays in the atmosphere. Therefore, a concentration of about 60 ppb of hydrogen or water inside the XENON1T detector could explain the observed excess, although the collaboration thinks the actual concentration should be ~100x lower.
Axions from the Sun
The more exciting interpretations of the signal are those that involve new physics and one of them would be the detection of solar axions. Axions are hypothetical particles, named after a brand of laundry detergent, and were postulated to solve a “fine-tuning” problem in particle physics. Axions are probably also the second most favorable dark matter candidate after WIMPs. However, dark matter axions have very small masses and need a totally different kind of detector technology to be searched for that involves microwave cavities. But axions with higher masses detectable by XENON1T can be produced in the sun through different processes such as the Primakoff effect. A statistical analysis shows that the data favors an axion signal with a significance of 3.2 sigma over the background model. If tritium is added to the background the axion signal is still favored but with a reduced significance of 2.1 sigma.
Neutrinos are not so Standard
The second interesting explanation of the signal comes from particles that we already know exist, namely neutrinos. Much like WIMPs, neutrinos are only weakly interacting and therefore show up very rarely in the detector. In fact, they were already considered in the background model but their contribution is minor. However, there are theories beyond the current Standard Model of particle physics that propose a higher interaction probability for neutrinos at low energies caused by a higher than usual magnetic moment of these particles. Fitting the data with this non-standard neutrino model gives a similar statistical significance as the axion model. But in this case, adding the tritium component reduces the significance to only 0.9 sigma.
Problems from Cooling Stars
One problem that should make us skeptical about the new physics interpretations of the signal is the fact that they are in tension with astrophysical observations. The properties of solar axions and the neutrino magnetic moment are constrained by studies about the cooling rate of stars which would lose additional energy through the emission of these particles. These constraints are in tension with the properties derived from the XENON1T data. On the other hand, particle physicists tend to not take astrophysical constraints too seriously because of the uncertainties involved in their models. Also, some astrophysical observations point towards an additional source of stellar cooling compatible with axions even if the parameters are different.
If the signal would be confirmed to originate from new physics the impact would be huge. It would be the first confirmation of physics beyond the Standard Model since the discovery of neutrino oscillations and therefore even outshine the discovery of the Higgs boson and the detection of gravitational waves. The good thing is that we should not have to wait too long until the situation is cleared up as the XENONnT, LZ, and PandaX-4T experiments are currently racing to build the next most sensitive WIMP detector. It looks like XENONnT will make the game as their detector is already being commissioned. With about three times as much xenon and a factor of ~6 lower background, the signal could be confirmed after only a few months of data collection. In the meantime, theorists have already started the publishing frenzy to come with new physics models that explain the excess, all hoping that this time the result will stick.
“it may still revolutionize physics” you mean like down is really up and fast is slow?
Up is down, black is white, and short is long.
Everything you know is wrong!
A bold statement from HaD! I was thinking maybe we got the arrow of time backwards. Uhm, I WILL be thinking we got the …..
And everything you thought was just so important doesn’t matter.
…or isn’t matter.
Okay, most of that went over my head.
obSoundEffect: “Whoosh!”
[youtube]TRqiFPpw2fY[/youtube]
We’re just the weird neighbour looking thru windows with a telescope. Even when the lights are off. Wait was that a windows phone in that dark window!
Probably not.
https://phys.org/news/2020-03-axiom-dark-numerical.html
https://journals.aps.org/prl/pdf/10.1103/PhysRevLett.124.101303
The axions that could possibly explain this excess are very different than the ones ADMX put limits on: they’re way heavier, and ADMX is really only searching for axion/photon coupling: that is, create wacko-big magnetic field, look for magic power coming out of nowhere from axions turning into photons. There’s no reason why axions *have* to interact in that parameter space.
The real constraints here come from astrophysics, because, well, stars are ridiculously huge balls of plasma, containing photons, electrons, and nuclei. If *any* kind of axion exists, it’s going to screw with how stars lose energy, and *that’s* hard to mess with.
“Axions are hypothetical particles, named after a brand of laundry detergent, and were postulated to solve a “fine-tuning” problem in particle physics.”
The ‘fine-tuning’ problem in question is the Strong-CP problem. A fine-tuning problem comes when you require nominally unrelated values to have some relationship between them in order to produce an observed value – one of the more commonly known ones is the cosmological constant, which if you associate it with zero-point energy in quantum field theory, should be Stupid Big (when it’s in fact quite small). So then you say “well, okay, there are quantum corrections to that”, but then those corrections also need to be very nearly exactly Stupid Big so (Stupid Big – Stupid Big = small). The bare value and corrections are unrelated, so there’s no reason why they should be very nearly exactly the same.
The strong-CP problem is, in my opinion, the wacko weirdest fine-tuning problem. CP’s easier to understand than people make it out to be: it’s really “matter acts different than antimatter” (although many places make it a bit more complicated than that, it’s really not – the confusion comes from how the matter/antimatter connection was viewed in the mid-20th century versus now). Both the strong force and the weak force *could* act differently between matter and antimatter. The weak force does. A little. It’s not very big, but it’s there.
The strong force doesn’t. At all. No CP violating effects have ever been seen in the strong force, down to far, far smaller levels than in the weak force. The “fine-tuning problem” part of the strong-CP problem is “why must this effect be so small?” but the reason I call it the “wacko weirdest” problem is that it’s not small. It’s currently *zero*.
Zero is much weirder than small, because you can generate “fine-tuning” solutions through ratios, instead of differences (i.e. Big/Stupid Big is small for a wide range of Stupid Big). You can’t do that if it’s zero. Zero is way harder to explain.
The awesome thing about axions is that unlike WIMPs (which, well, do… nothing) axions *actually screw around with easily observable physics* – it means that Maxwell’s Laws are all slightly wrong, and Weird Stuff might happen at very high electromagnetic fields. And humans are *really good* at generating/manipulating really big electromagnetic fields.
Outta ALL the gin joints, you walk into this one – your post neither stupid nor small. Such a pleasant change.
Even as an EE (doesn’t matter to me, the equations are all good enough for power calcs hahaha ), that’s been my hope. I’ve seen techniques for creating crazy strong but very tiny magnetic fields (tiny but fierce! ) that I want to see something cool be done with.
So does anyone else think that Dark Matter may turn out to be the 20th century’s Luminiferous Aether?
yeah.
No, but seemingly a lot of people do. Dark matter has many different lines of very strong evidence though, Luminiferous Aether didn’t have any lines of evidence and was invented to solve a problem that it turns out didn’t exist.
Wasn’t dark matter invented to address the problem of “missing mass” in the universe as observed as compared to theory?
The disparity isn’t between observation and theory, it’s between two different observations that we have high confidence in. You can look at a galaxy and determine it’s mass from the light/radio radiation received, or you can look at how it moves, which tells you how much gravity it’s under and therefore how much mass there must be. There’s a *lot* more gravity than can be accounted for in the direct measurements.
So either gravity behaves differently at those scales and gets a lot stronger (probably unlikely), or there’s more matter there that we can’t detect via traditional methods. Hence it’s ‘dark’.
It’s more than that, actually, and the “dark” at this point means “couples weakly to light.” At first, when the only observations that suggested dark matter were galaxy clusters/galaxy rotation, the “dark” just meant “not glowing,” and it could’ve been explained by any number of things, including tons and tons of small brown dwarfs/black holes/etc., which generically got termed “massive compact halo objects” (MACHOs, in contrast to WIMPs, because physicists think they’re comedians). Observations eventually ruled those out, but the real death knell for dark matter being “normal” came from the cosmic microwave background (CMB), which makes things *much* weirder.
See, the CMB is the last glowing remnant of light from when the Universe was a big ionized ball of plasma. Because it was ionized, all the matter and photons coupled together and acted as one thing, much like the Sun: plasmas are opaque, gasses are transparent. But eventually the Universe cooled, and stopped being a plasma. But while it *was* a plasma, there were sound waves propagating in it, and like anything in a fixed size container, there are fundamental modes of those waves.
Because the universe started off hot and cooled down, it’s like an open bottle (fixed one end, open the other) – first fundamental mode is a quarter-wavelength. All the “odd” modes (1/4, 3/4, 5/4, etc.) correspond to “hot in the beginning, cool at the end.” All of those imply the matter is acting “normally” – when it compresses, it gets hot (emits light) and expands again.
But if you look at the actual power spectrum of the CMB, there’s a ton of power in *even* modes. These imply that the matter went hot, cold, hot again: which *isn’t* matter working normally. There was something acting *even before the first stars were made* to compress matter *more* than it naturally would if it was just coupled to light.
Same problem (too much gravity), but much bigger issue, because it means there was too much gravity *very* early on. Before there were stars, and before there were even atoms, back when the Universe was (relatively) tiny – at recombination our Universe was only ~14 Mpc in radius.
That’s why lambda-CDM (dark energy + cold dark matter) became the ‘standard model’ for the Universe: because it explains a ton of different observations with one basic model. From before stars were born all the way to the anomalous rotation and motion we see now.
The downside to the model is that it has two parts that we have no particle physics model for (dark energy and cold dark matter). But, sadly, there’s no reason the Universe has to be nice to us: these two effects don’t have to have any effects we can screw around with in labs.
NASA told us there were eddies detected in the Aether we don’t have a couple of years back.
“Eddies,” said Ford, “in the space-time continuum.”
“Ah,” nodded Arthur, “is he. Is he.”
“What?” said Ford.
“Er, who,” said Arthur, “is Eddy, then, exactly, then?”
42
Welcome to pop science versus real science. We *don’t* have a ‘Newtonian relativistic’ aether. We might have a quantum relativistic one, though. Over the years, calling it an “aether” fell very much out of favor, but Dirac was publishing articles about a possible quantum-relativistic aether in the 50s, and that expanded into the idea of a possible superfluid vacuum.
“Luminiferous Aether didn’t have any lines of evidence and was invented to solve a problem that it turns out didn’t exist.”
That’s not really true. The problem wasn’t the idea that light needs a medium to propagate in, and in fact, it isn’t entirely untrue, as the Higgs superfluid in some sense acts as the medium that allows light to propagate – if it were somehow possible to alter the vacuum Higgs field, light would scatter off of it like rocks in a pond. Plus the entire idea that space is somehow “nothing” isn’t really right in any case. Which is funny, because one of the things that bothered people about the aether was that it was some “stuff” that filled the universe, everywhere, but you couldn’t feel it. Papers back then had arguments about where the aether could penetrate and where it couldn’t. Funny enough, nowadays, we don’t have any problem with things like that.
And it *did* solve problems: stellar aberration was explained by the relative motion of Earth and the aether. Problem was it didn’t seem to jibe with other ideas that people had about the way the aether worked (differences in index of refraction, etc.). But the really fatal problem was that none of the ideas worked with the Michelson-Morley experiments, which actually showed that what was wrong was *Newtonian relativity*.
In fact they *could’ve* kept the idea of the aether around, and the Higgs particle might’ve even be eventually called the “aether particle”, but there didn’t seem to be a *reason* to keep it around. Lorentz even made exactly this argument (that the idea of the aether might eventually come back, because it wasn’t really the problem) although it took so long to be understood that the aether had a bad reputation in public forums by then.