When I was a kid we used to go to a place we just called “The Book Barn.” It was pretty descriptive, as it was just a barn filled with old books. It smelled pretty much like you’d expect a barn filled with old books to smell, and it was a fantastic place to browse — all of the charm of an old library with none of the organization. On one visit I found a stack of old magazines, including a couple of Popular Mechanics from the late 1940s. The cover art always looked like pulp science fiction, with a pipe-smoking father coming home from work to his suburban home in a flying car.
But the issue that caught my eye had a cover showing a couple of rugged men in a Jeep, bouncing around the desert with a Geiger counter. “Build your own uranium detector,” the caption implored, suggesting that the next gold rush was underway and that anyone could get in on the action. The world was a much more optimistic place back then, looking forward as it was to a nuclear-powered future with electricity “too cheap to meter.” The fact that sudden death in an expanding ball of radioactive plasma was potentially the other side of that coin never seemed to matter that much; one tends to abstract away realities that are too big to comprehend.
Things are more complicated now, but uranium remains important. Not only is it needed to build new nuclear weapons and maintain the existing stockpile, it’s also an important part of the mix of non-fossil-fuel electricity options we’re going to need going forward. And getting it out of the ground and turned into useful materials, including its radioactive offspring plutonium, is anything but easy.
Lixiviants and Leachates
Despite its rarity in everyday life, uranium is surprisingly abundant. It’s literally as common as dirt; stick a shovel into the ground almost anywhere on Earth and you’ll probably come up with a detectable amount of uranium. The same goes for seawater, which has about 3.3 micrograms of uranium dissolved in every liter, on average. But as with most elements, uranium isn’t evenly distributed, resulting in deposits that are far easier to exploit commercially than others. Australia is the winner of this atomic lottery, with over 2 million tonnes of proven reserves, followed by Kazakhstan with almost a million tonnes, and Canada with 873,000.
While most of the attention uranium garners has to do with the properties of its large, barely stable nucleus, the element also participates in a lot of chemical reactions, thanks to its 92 electrons. The most common uranium compounds are oxides like uranium (IV) oxide, or uranium dioxide (UO2), the main mineral in the ore uranite, also known as pitchblende. Uranite also contains some triuranium octoxide (U3O8), which forms when UO2 reacts with atmospheric oxygen. The oxides make up the bulk of commercially significant ores, with at least a dozen other minerals including uranium silicates, titanates, phosphates, and vanadates being mined somewhere in the world.
Getting uranium out of the ground used to be accomplished through traditional hard-rock mining techniques, where ore is harvested from open-pit mines or via shafts and tunnels running into concentrated seams. The ore is then put through the usual methods of extraction that we’ve seen before in this series, such as crushing and grinding followed by physical separation steps like centrifugation, froth flotation, and filtration. However, the unique chemical properties of uranium, especially its ready solubility, make in situ leaching (ISL) an attractive alternative to traditional extraction.
ISL is a hydrometallurgical process that has become the predominant extraction method for uranium. ISL begins by drilling boreholes into an ore-bearing seam, either from drill rigs on the surface or via tunnels and shafts dug by traditional mining methods. The boreholes are then connected to injection wells that pump a chemical leaching agent or lixiviant into the holes. For uranium, the lixiviant is based on the minerals in the ore and the surrounding rock, and is generally something like a dilute sulfuric acid or an aqueous solution of sodium bicarbonate. Oxygen is often added to the solution, either via the addition of hydrogen peroxide or by bubbling air through the lixivant. The solution reacts with and solubilizes the uranium minerals in the ore seam.
ISL offers huge advantages compared to conventional mining. Although uranium is abundant, it’s still only a small percentage of the volume of the rock bearing it, and conventional mining requires massive amounts of material to be drilled and blasted out of the ground and transported to the surface for processing. ISL, on the other hand, gets the uranium into aqueous solution while it’s still in the ground, meaning it can be pumped to the processing plant. This makes ISL a more continuous flow process, as opposed to the more batch-wise processing methods of conventional mining. Plus, the lixiviant can be tailored to the minerals in the ore so that only the uranium is dissolved, leaving the rock matrix and unwanted minerals underground.
Reacting With Hex
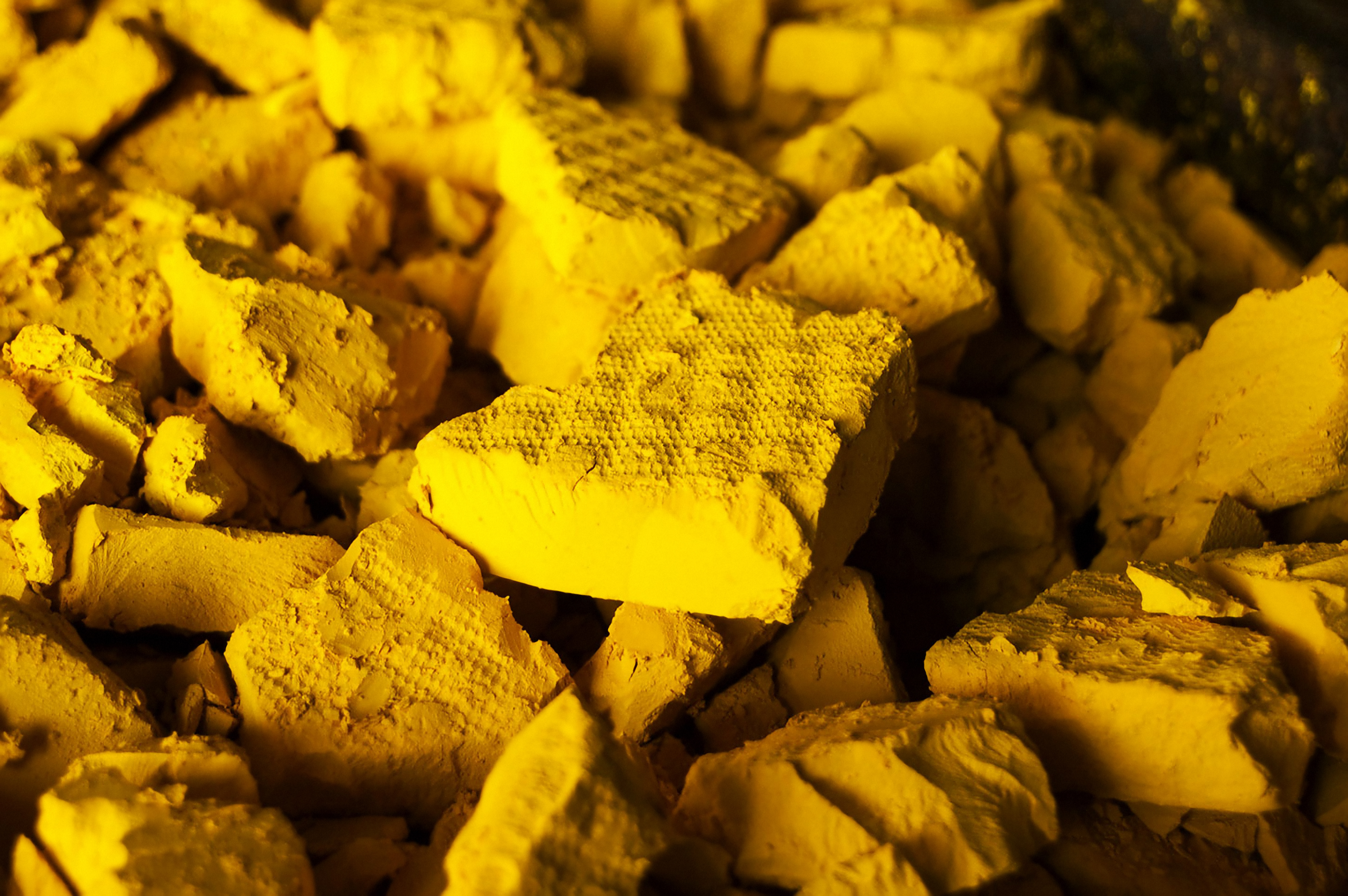
Uranium dioxide (UO2) is the primary endpoint of uranium refinement. It’s a dark gray powder; the so-called “yellow cake” powder, which is also produced by chemical leaching, is an intermediate form in uranium processing and contains a mix of oxides, particularly U3O8. Natural uranium oxide, however, is not especially useful as a nuclear fuel; only a few reactors in the world, such as the Canada Deuterium Uranium (CANDU) reactor can use natural uranium directly. Every other application requires the uranium dioxide to be enriched to some degree.
Enrichment is the process of increasing the concentration of the rare fissile isotope 235U in the raw uranium dioxide relative to the more abundant, non-fissile isotope 238U. Natural uranium is about 99.7% 238U, which can’t sustain a chain reaction under normal conditions, but with three fewer neutrons in its nucleus, 235U is just unstable enough to be fissionable under the right conditions.
Unlike refining, which takes advantage of the chemical properties of uranium, enrichment is based on its nuclear properties. Separating one isotope from another, especially when they differ by only three neutrons, isn’t a simple process. The vast majority of the effort that went into the Manhattan Project during World War II was directed at finding ways to sort uranium atoms, and many of those methods are still in use to this day.
For most of the Cold War period, the principal method for enriching uranium was the gaseous diffusion method. Uranium oxide is first turned into a gas by reacting it with hydrofluoric acid to form uranium tetrafluoride, which is then treated with fluorine to first yield uranium pentafluoride and finally uranium hexafluoride:
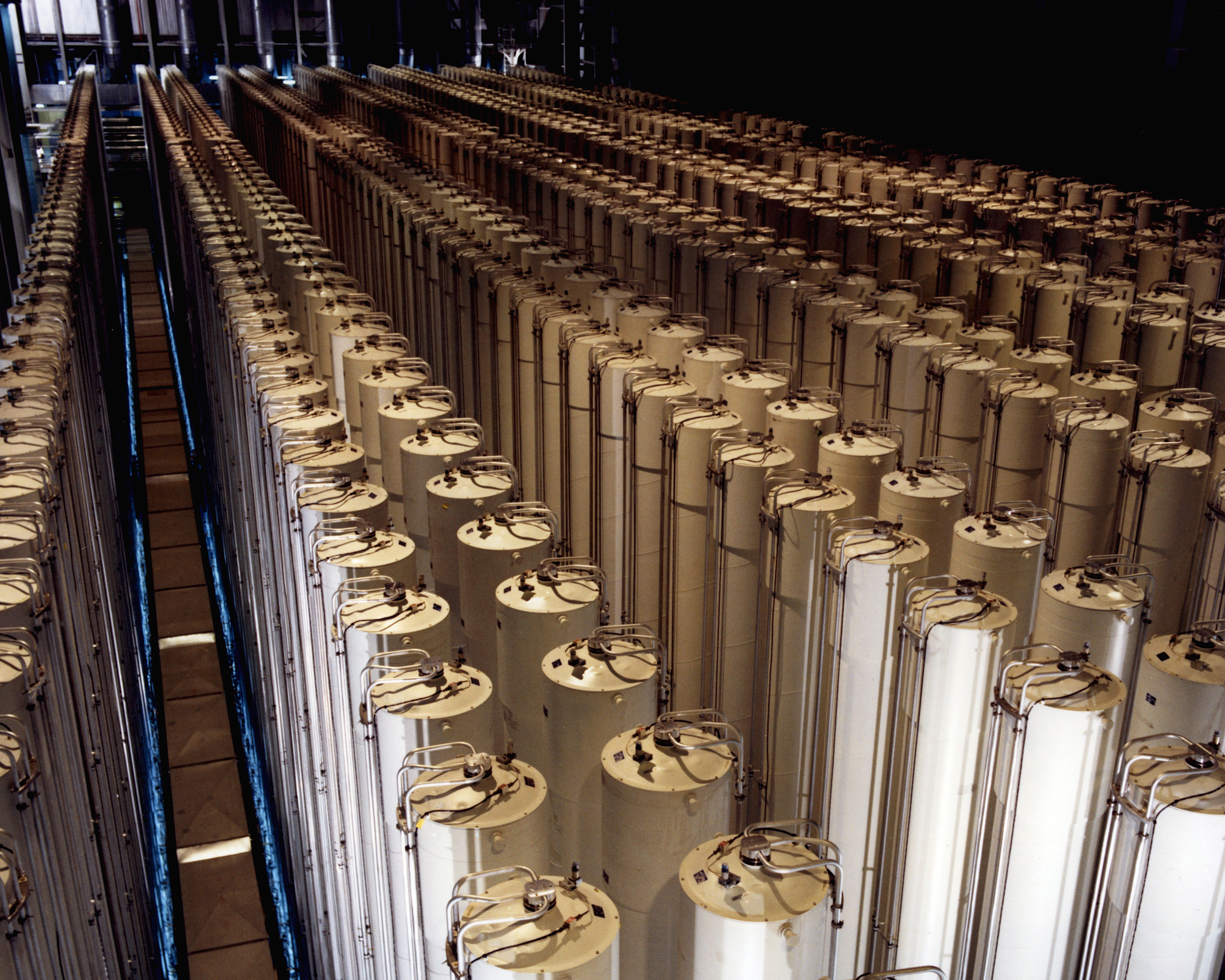
The highly volatile, incredibly corrosive uranium hexafluoride gas, or hex, is pumped at high pressure into a pressure vessel that contains a semi-permeable separator made from sintered nickel or aluminum. The pore size is tiny, only about 20 nanometers. Since the rate at which a gas molecule passes through a pore depends on its mass, the slightly lighter 235UF6 tends to get through the barrier faster, leaving the high-pressure side of the chamber slightly depleted of the desirable 235U6. Multiple stages are cascaded together, with the slightly enriched output of each stage acting as the input for the next stage, eventually resulting in the desired enrichment — either low-enriched uranium (LEU), which is in the 2-3% 235U range needed for civilian nuclear reactor fuel, or high-enriched uranium (HEU), which is anything greater than 20% enriched, including the 85-90% required for nuclear weapons.
These days, gaseous diffusion is considered largely obsolete and has given way to gas centrifugation enrichment. In this method, gaseous hex is pumped into a tall, narrow cylinder spinning in a vacuum at very high speed, often greater than 50,000 revolutions per minute. The heavier 238UF6 is flung against the outer wall of the centrifuge while the lighter 235UF6 migrates toward the center. The slightly enriched hex is pumped from the center of the centrifuge and fed into the next stage in a cascade, resulting in the desired enrichment. The enriched hex can then be chemically converted back into uranium dioxide for processing into fuel.
Made, Not Found
Unlike any of the other elements we’ve covered in the “Mining and Refining” series so far, plutonium is neither mined nor refined, at least not in the traditional sense. Trace amounts of plutonium do exist in nature, but at the parts per trillion level. So to get anything approaching usable quantities, plutonium, the primary fuel for nuclear weapons, needs to be synthesized in a nuclear reactor.
The main fissile isotope of plutonium, 239Pu, is made by bombarding 238U with neutrons. Each atom of 238U that absorbs a neutron becomes 239U, a radioactive isotope with a half-life of only 23.5 minutes. That decays via beta radiation to neptunium-239 (239Np), another short half-life (52 hours) isotope that decays to 239Pu:

The process of creating 239Pu from uranium is called “breeding.” From the look of the reaction above, it seems like a civilian nuclear reactor, with its high neutron flux and fuel rods composed of about 96% unenriched uranium, would be the perfect place to make plutonium. There are practical reasons why that won’t work, though, and it has to do with one little neutron.
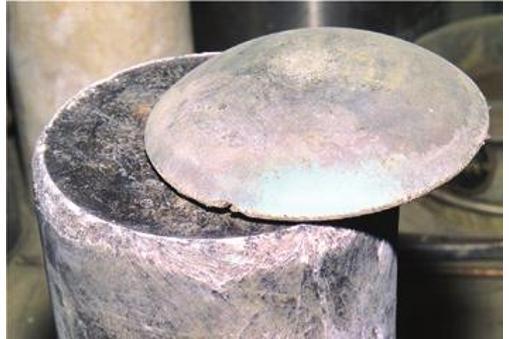
Plutonium isn’t really enriched the way that uranium is. Rather, plutonium is graded by the amount of 240Pu it contains; the lower the concentration relative to 239Pu, the higher the grade. That’s because 240Pu tends to undergo spontaneous fission, releasing neutrons that could pre-detonate the plutonium core of the bomb before it’s completely imploded. Weapons-grade plutonium has to have less than 7% 240Pu, and the longer the reaction is allowed to continue, the more it accumulates. Weapons-grade plutonium can only cook for a couple of weeks, which means a civilian reactor would need to be shut down far too often for it to both generate power and synthesize plutonium. So, special production reactors are used to create fissile plutonium.
Once the fuel rods in a production reactor are finished, the plutonium is chemically separated from any remaining 238U and other contaminating fission byproducts using a long, complicated process of extraction. One such process, PUREX (plutonium uranium reduction extraction), uses nitric acid and a combination of organic solvents like kerosene to dissolve the uranium, plus aqueous solvents and reducing agents to solubilize the plutonium. Plutonium dioxide can then be reduced to metallic plutonium, for example by heating it with powdered aluminum. The resulting metal is notoriously difficult to machine, and so is often alloyed with gallium to stabilize its crystal structure and make it easier to handle.
“Weapons-grade plutonium can only cook for a couple of weeks, which means a civilian reactor would need to be shut down far too often for it to both generate power and synthesize plutonium. So, special production reactors are used to create fissile plutonium.”
Not if you use RBMK, which can be refueled without shutting down the reactor. You get cheap power and weapons-grade plutonium… oh, and sometimes Exclusion Zones if your reactor is run by yet another corporate SCRUM master who consider engineers to be the main obstacle in “delivering the project”.
—
If you’re into those things, Jerzy Kubowski wrote two interesting books: “Nuclear Power Plants” and “Nuclear Weapons”.
If online refueling is the only requirement then you could, in theory, use a CANDU as well.
India did use a CANDU to get its plutonium.
Two main things to add: Civilian reactors can make weapons grade material , but it needs either design for live fuel changing (RMBK), or is pretty obvious from monitoring it. If you’re a nation and you want nuclear weapons, the economic argument falls out the window however!
Monitoring it is (among other things) exactly what IAEA/ITAR safeguards do _now_ – “is country x shutting down it’s reactors every ~2 weeks?” “does the satellite show trucks leaving the NPP every 2 ish weeks and driving to military bases”. “does this waste contain the right ratio of U:P239:P240?” “can we account for all the uranium going in, and waste going out?”. (and proactively, “is this design deliberately bad for Pu production”).
The other thing is that a lot of the early nuclear facilities were specifically designed to make plutonium (with power as an extra), in the rush to get atomic weapons, e.g. UK (Windscale, Magnox), the RMBK. Can second reading up on this fascinating history – the 1940s-70s nuclear race has impacts on a lot of countries today. “Atomic accidents” by James Mahaffey was interesting.
Of course, deliberately making Pu239/240 rich fuel gets you a lot (140x?) more energy per tonne of U239, and that’s a breeder reactor…but then you can see how much more regulated they are since you could just close your borders, block inspections, and make yourself a world player/rogue state/atomic nation. (Oh, and fast reactors too – you can burn up “spent” U fuel, and massively reduce down more dangerous waste by _breaking apart the atoms with neutrons_).
This is one of the article’s errors: “cooking for a couple of weeks” (really about a month) yields a majority of Pu-238, the world’s favorite RTG (RadioIsotope Thermal Generator) fuel. To get a majority of Pu-239 requires a few months, typically around 4. More than that (like in power reactors) and you get a lot of Pu-240 which likes to spontaneously fission and makes the mix unusable for weapons as well as quite dangerous (to people) from all the neutron flux.
This is also the region yielding a lot of Am-241 which CAN be chemically separated and is the main isotope used in smoke detectors as well as being seriously looked at for deep space RTGs; unlike Pu-238, Am-241 has a small amount of weak but easily shieldable gammas (~55kV from memory) which is why an unopened smoke detector has little to no detectable radiation from a pancake GM tube.
Uranium and plutonium
There’s some metals heavier than lead
But worth more than gold atom per atom
If you can mine 2 pounds of it
You can retire permanently
Roughly 15-20 million per kilo
Plutonium cost more since you’ll need a nuclear reactor and a supply of uranium already
nope, Pu239 is several times cheaper compared to U235…you seriously underestimate just how much of a pain isotope separation is, especially when starting at 0.7%
Are you accounting for the man hours, and energy and infrastructure costs?
You still need the uranium or other heavly fissles which are already costly
Yep, it may well be the right time to start drilling through the floor of my basement. Who knows what might be found? Drill cores will be used to build a rockery in the garden.
Good chance you’ll find some Radon, which is still heavier than lead but you’ll pay to have it taken away.
errr ….. radon is a gas.
But atomically it’s heavier than lead
Yep, if you are in the US and you succeed in mining 2 pounds of it, you’ll get to permanently retire at ADX Florence.
No they’ll “offer” to buy the land from you and pay you for the uranium
And you’ll live a rich sumbitch
Then it becomes federal land owned by department of energy
Thru LANL
LANL for u civilian people
Los Alamos Nuclear Laboratory
Los Alamos National Laboratory
3.3 ug/l in seawater, hunh? 3.3 mg/tonne. Is that a useful amount?
A mid-size city uses about 100 kilotonnes of water per day. If provided by desalination from ocean water, the effluent would contain the separated 330 grams of uranium.
A reactor burns about 24 g of (natural, unenriched) uranium per MWh, so that 330 g/d would only produce 14 MWh/d, just 570 kW.
So, that kind of sucks. The uranium produced from a city’s worth of water usage would only supply enough electricity for a single apartment building.
On the other hand, the oceans contain a million times the amount of uranium reserves of the top three countries combined.
” the fissioning of 1 gram of fissile material yields about 1 megawatt-day (MWd) of heat energy.”
Assuming your reactor can burn (or you can recycle) all the uranium into fissile material, you would actually get 330 MW out of it. If you count just the once-through cycle that burns 2-4% then it would be about 10 MW.
1 gram of fissionable materials produces about 1 Megawatt-days of energy (heat).
Correct. Multiply that by the 0.7% concentration of the fissionable isotope, multiplied by the thermal efficiency of the power plant, and you get the same number I quote.
You can breed and recycle the fuel.
Natural uranium (99.3% U-238, 0.7% U-235) in fast breeder reactor: 86,000 MJ/g = 24 MWh/g
Adjusting for efficiency it comes out at roughly 100 MW for 330 grams per day.
Even with France’s recycling program and India’s efforts at commercial breeders, are there *any* fuel breeding and reprocessing programs that have any hope of getting anywhere near that kind of process efficiency?
Hard to say, but I would hazard that you can get much more than 0.7%
A conventional light water reactor has a conversion ratio of about 0.6, so they do make more fissile fuel as they run, resulting in a fuel burn of more than the 0.7% that’s initially in the fuel. Breeder reactors have a conversion ratio up to 1.2 so they could in theory turn all the uranium into fuel.
Apparently the Indian reactor had 1.4% more fissionable fuel after five years running than it started with.
The reason why it can’t go forever is the accumulation of “poison” elements which impede the reaction, so you have to take the fuel out and reprocess it to remove the side products.
It seems like that method of mining would only work if the deposit was in a significantly porous matrix.
And while we’re on the subject.. anybody care to weigh in on groundwater contamination?
If you are interested in this topic, there is a place called Stráž pod Ralskem (CZ), where this kind of “mining” has been in operation. The environment damage appears to be a real problem and it is really hard to stop the operation there. (For more info see http://podzemi.solvayovylomy.cz/histhor/lokality/straz/straz.htm – in Czech, but google translate it reasonable well.)
To get useful amounts of U235, you must have lots of money, lots of time, and/or lots of electricity. Choose at least 2.
Could you use the “Leech Mining” techniques on other metals, like Lithium? Sure would be nicer looking than huge stripe mines.
Apparently there is something called Direct Lithium Extraction, which is not exactly the same as In Situ Leaching described here but is similar in that the brine containing the lithium is pumped out of the earth, the lithium extracted, and then brine pumped back into the earth (rather than into evaporation pools). IEEE Spectrum article: https://spectrum.ieee.org/direct-lithium
There is a direct lithium extraction plant already being constructed at the Salton Sea (about 100 km from the coast near Los Angeles, California).
please don’t write chemical or nuclear reactions with italics. it is not right. also units… don’t italicize them.
+1
Are there any recommendations on books about mining / metallurgy? The inventions and developments in the Cold War era are quite interesting.
There was also “thermal gradient isotope separation”, I wonder why that fell out of favour. Too slow?
Too unstable
Ever heard of the natural fission reactor in Oklo, Gabon ?
https://fr.wikipedia.org/wiki/R%C3%A9acteur_nucl%C3%A9aire_naturel_d%27Oklo
https://en.wikipedia.org/wiki/Natural_nuclear_fission_reactor
This tends to prove that human beings do not have the exclusivity of nuclear reactors !